Negative Emissions
This chapter shows a just, socially accepted and environmentally compatible way of including negative emissions into the mitigation efforts to reach the goal of net 0 emissions by 2030.
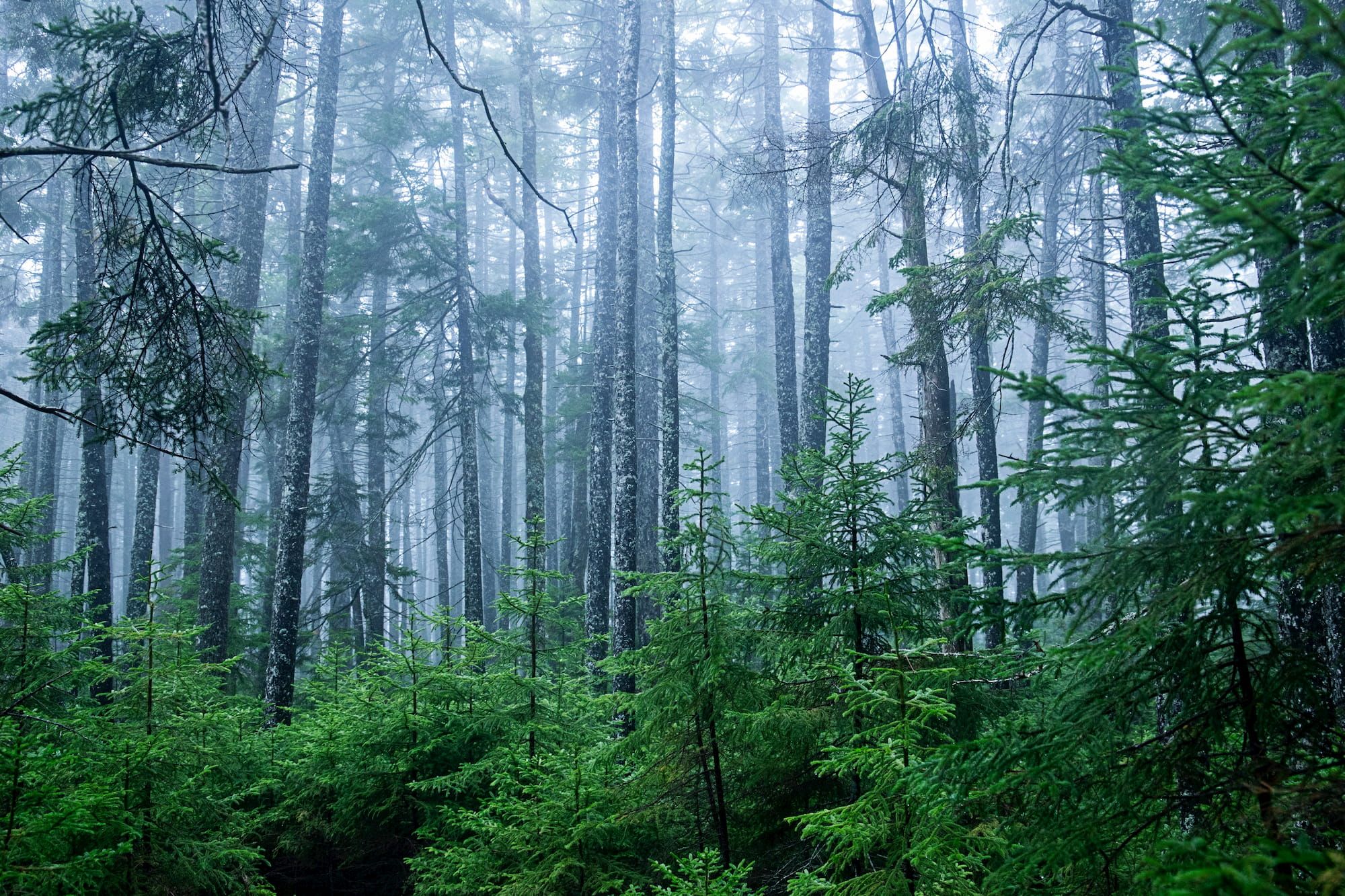
You are reading the full version of the Climate Action Plan. Do you just want to read the Executive Summary? Click here for the Executive Summary:
Some abbreviations are used in this chapter. You can find explanations of all abbreviations in the glossary.
Current Situation
Introduction
In the Special Report on Global Warming of 1.5 °C, the IPCC collected all emissions pathways published in scientific literature compatible with the 1.5 °C target. All of the 90 climate scenarios require the large-scale utilization of Negative Emissions Technologies (NETs, technologies to perform Carbon Dioxide Removal, CDR), starting between 2020-2030 and removing on average 32% of the 1990’s annual emissions by 2050. NETs are used to extract CO2 from flue gases or directly from the atmosphere and store the CO2 securely away from the atmosphere for centuries to come. NETs are no alternative or excuse to mitigation but a needed addition if respective carbon budgets are exceeded. The technical, permanent removal of CO2 is energy intensive and expensive, therefore mitigation will be the economically most viable option in many cases. There exists a vast diversity of NETs, some are purely technological, others use plants or algae to extract the CO2 as a capturing method. The global potential storage capacity is assessed to be more than 2000 Gt CO2 (IPCC 2018). All NETs are based on natural analogues, for example CO2 is stored in geological formations (sandstone sealed with clay and marl) in Montmiral in France since 15 million years (Pearce et al. 2004) or weathering of stone has been the main CO2 sink for the past 850’000 years.
Most likely, there will not be the single NET to be implemented globally, but rather selected NETs based on regional conditions. What all NETs have in common is their low level of deployment in 2020 and the subsequent lack of the economy of scale leading to high costs.
This chapter discusses the socio-economic effects of NETs, summarizes the most established NETs, their potential in Switzerland, and their price. In Switzerland, the most promising NETs are Biochar, Direct Air Carbon dioxide Capture and Storage (DACCS, if financed by Switzerland but securely implemented abroad), Carbon dioxide Capture and Storage (CCS) in Swiss cement- and waste incinerator plants, and soil carbon content/sequestration. These technologies are key for Switzerland to meet its share on achieving the 1.5 °C target. Hence, its prompt incorporation into any future emissions strategies is vital.
Scientific Background
The need for prompt action is evident at least since the time of the first IPCC report in 1990 and has consolidated ever since. Research has shown that global warming is roughly proportional to the total amount of carbon dioxide released into the atmosphere (of which a substantial fraction remains in the atmosphere for thousands of years (Knutti and Rogelj 2015). Independently of the global mean temperature to be achieved (e.g. +1.5, +2, +4.5 °C) the earth keeps warming if CO2 emissions continue such that CO2 in the atmosphere accumulates (emissions exceed net zero) (Zickfeld et al. 2009).
The near-proportionality between cumulative CO2 emissions and global temperature also makes it possible to estimate the remaining carbon budget: the total amount of anthropogenic carbon dioxide that can still be emitted into the atmosphere while holding the global average temperature increase to the limit set by the Paris Agreement (Rogelj et al. 2019). This already shows the need for negative emissions, as eliminating all anthropogenic CO2 emissions seems highly unrealistic.
Negative emissions are realized when carbon dioxide is removed from the atmosphere and sequestered for a long period, through technological or biological processes. An example for a technological process is the capture of CO2 from ambient air done by Climeworks in Switzerland, and storing it permanently in deep geologic formations. This technology is, apart from large scale funding, ready for implementation. An example for a biological process is the removal of CO2 from the atmosphere by trees and the storage as wood. The wood can be used as construction material to avoid re-emitting captured GHGs back into the atmosphere. Currently, Switzerland exhibits only negative emissions in the sector LULUCF (Land use, land use change and forestry).
It should be highlighted that negative emissions are not a substitute for other mitigation efforts, but a completion. The goal is not to develop large scale carbon dioxide removal, but rather to stay within the extremely small remaining carbon budget to reach the 1.5 °C goal.
While negative emission technologies can compensate emissions and help to reach net zero, it is often assumed that after reaching net zero emissions it will be required to remove more CO2 from the atmosphere than is actually brought in, in order to limit global warming to 1.5 °C. The term for this scenario is called ‘net negative emissions’ (in contrast to NETs - negative emission technologies).
Negative emissions in the IPCC scenarios

The total NET (technologies to perform Carbon dioxide removal, CDR, as referred to in the IPCC reports) deployment levels within the different 1.5 °C compatible scenarios with no or limited overshoot cover between 100 and 1000 Gt CO2 until the year 2100 (IPCC 2018) central role of NETs in the latest IPCC reports is a consequence of previous failures in global climate protection (FOEN 2019e).
All of the scenarios compatible with the 1.5-degree goal do not only use NETs to reach net zero, but after 2050 also to achieve total net negative emissions. I.E. they exceed the remaining carbon budget on the assumption that the exceeded amount will be taken out of the air by future generations.
The longer the delay in reducing CO2 emissions towards zero, the larger the likelihood of exceeding 1.5°C, and the heavier the implied reliance on net negative emissions after mid-century to return warming to 1.5°C (IPCC 2018).
An overshoot in atmospheric GHG concentrations for a few decades results in irreversible loss of biodiversity, e.g. a fish, which has died in hot water, cannot be simply brought back to life by lowering the water’s temperature. Also, the overshoot might trigger tipping points, which upset the global ecosystems and make a return to the original state much harder.
A linear reduction of the global CO2 emissions from 2020 until 2050 would exceed the remaining carbon budget for a 67%-chance to reach the 1.5 °C goal by about 260 Gt CO2. Thus, the 1.5 °C goal, together with the goal net zero until 2050, always means a heavy reliance on net negative emissions.
For high (per capita) emitting countries like Switzerland, which have already used a large amount of the remaining carbon budget starting in 2018, net zero until 2050 is even less compatible with the 1.5 °C goal. Distributing the global remaining carbon budget from 2019 on (320 minus 40 Gt CO2) equally under all word citizens would give Switzerland (annual consumption based CO2 emis-sions of about 100 Mt CO2) a remaining carbon budget of about 205 Mt CO2 ((280/world popula-tion)*swiss population - swiss emissions since 2019). This means a linear reduction from now until 2024 is required from all Swiss residents, and not 2050, in order to stay within the 1.5 °C carbon budget.
There are enormous problems associated with reliance on net negative emissions.
One problem of the scenarios featured in the IPCC SR1.5 is that discounting rates of integrated assessment models (IAMs) were too high. “To ensure inter-generational equity and be coherent with cost-benefit analysis normative choices, we suggest that IAMs should use lower discount rates than the ones currently adopted” (Emmerling et al. 2019).
But the main problem might be that the models produce lowest-cost-scenarios, independent of the question whether these costs will be paid (Bednar et al. 2019). If more CO2 should be taken out of the atmosphere than put into, the possibility of NETs financed by the polluters ceases to exist. This is the most important difference between the notions ‘negative emissions’ and ‘net negative emissions’.
Furthermore, there is no guarantee that all countries will participate in net CO2 removal. On the contrary: not participating might be attractive. And countries who participated might punish the free riders by declining cooperation.
In addition, limits to our understanding of how the carbon cycle responds to net negative emissions increase the uncertainty about the effectiveness of carbon dioxide removal to decline temperatures after a peak (IPCC 2018).
Last but not least, reliance on future negative emissions are used to justify delays in mitigation efforts. The promise of future and cost-optimal NETs is more politically appealing than the prospect of developing policies to deliver rapid and deep mitigation now (Anderson and Peters 2016).
To understand the fundamental difference between the promise of negative emissions and the implementation of negative emissions, consider the result of the following two rules:
- “What you emit today will be removed later”.
- “You have to remove today what you emit today.”
The first rule means further delay in climate mitigation, the second rule means net zero emissions and at the same time provides an incentive to avoid emissions completely. Our policies give an approach how the second rule can be made enforceable as fast as possible.
All in all, the reliance on future net negative emissions, and also the promise of future negative emissions (not necessarily net negative), must be seen as an intergenerational deception. Therefore, we have to strictly reject overshoots – a period of warming over 1.5 degrees – as this might as well trigger tipping points in the climate system and go beyond other irreversible thresholds relating to, for example, biodiversity loss. We should rather try to stay within the global remaining carbon budget left to reach the 1.5 °C goal: Rapid emissions reductions and carbon capture from point sources as well as application of NETs as soon as possible.
Another possible point of view is, that we will need net negative emissions either because Switzer-land has already exceeded its fair share of the global remaining carbon budget because of all historic emissions, or, for the case that all measures won’t succeed and we will exceed the remaining budget of approx. 206 Mt CO2.
In our chapter we do not further consider such cases with net negative emissions for the following reasons:
- The problems arising with net negative emissions described above show that we should not plan to have net negative emissions.
- The first and most important challenge is to reach net zero as fast as possible. If afterwards net negative emissions will be declared necessary, we will have to face the difficulties described above.
- While trying to convince the population of the need for negative emissions, we already face difficulties without including all historic emissions. Thus, we try to focus on the current goal to reach net zero as fast as possible.
Need of Near-Term Implementation of Negative Emissions
This chapter does not promote any promise of the possibility to remove current emissions later. It rather explains why we have to make negative emissions ready as fast as possible in order to facilitate real time removal of emissions that cannot be eliminated fast enough.
If Switzerland is to provide a minimally fair share to contain the global temperature increase as agreed in Paris, it must keep its remaining carbon budget below less than 265 Mt CO2 calculated in chapter political and economic structure (205 Mt CO2 if the global remaining carbon budget is distributed equally under all world citizens already from 2019 on), and reduce its non-CO2 greenhouse gases to net zero until 2030 for the following reasons:
- Switzerland produces most of its consumption-based emissions abroad (BFS 2018), where it cannot directly influence the production and transport process.
- Switzerland has a relatively low-carbon intensity, due to its low-carbon total primary energy supply (supported by 26% of renewable sources of energy, with 13% hydro and 23% nuclear) and its economy dominated by the service sector, with about a quarter share of GDP from industry where the manufacturing sector and energy-intensive process industries play a miniscule role (IEA, n.d.) Switzerland thus faces less difficulties to become carbon neutral than other countries.
- Switzerland's high gross domestic product per capita might lead to a bigger responsibility.
- Switzerland finances greenhouse gas emissions abroad. (see chapter international climate finance and collaboration and chapter financial sector.)
- Switzerland has relatively high historic per-capita-emissions. Thus, one could distribute the global CO2-Budget from an earlier point in history, such that Switzerland's remaining share of the global carbon budget from 2020 on would be much smaller.
Also, it is clear that some greenhouse gas emissions are inevitable, even with an enormous effort. None of the non-CO2 forcers reach zero in the 1.5 °C IPCC scenarios until 2100. If these GHG emissions are not eliminated, they have to be counterbalanced by negative emissions. Examples are remaining nitrous oxide and methane emissions by agriculture or remaining carbon emissions by cement production and aviation.
Regarding the very small remaining greenhouse gas budget, it is irresponsible to keep postponing negative emissions on the assumption that our efforts to reduce our GHG emissions will succeed and GHG emissions will come down fast enough. As opposed to this, implementing negative emissions alongside all other mitigation efforts accelerates the greenhouse gas emissions reduction, and negative emission technologies (NETs) in particular accelerate the transition to a renewable energy supply.
Another reason for a fast implementation of NETs is, that in global comparison, Switzerland has already a very strong position in various carbon dioxide removal approaches, and should therefore already promote a corresponding structural change today in order to establish a leading position (first mover advantage) (Christoph Beuttler Jens Leifeld, Martin Schmid et al. 2019)
EASAC (2018) writes: “Despite the limitations of NETs (negative emission technologies), halting increases in the concentration of GHGs in the atmosphere remains a race against time, and humanity will require all possible tools to limit warming to within Paris Agreement targets.” In this Chapter we will show which kinds of negative emissions can be counted as “possible tools” for Switzerland.
In our chapter we do not further consider cases with net negative emissions as the problems arising with net negative emissions described earlier in the chapter show that we should not plan to have net negative emissions and the most important challenge is to reach net zero as fast as possible.
Concerns and Misunderstandings about Negative Emissions
Nevertheless, there are concerns and misunderstandings about negative emissions. Being aware of them helps us to develop a concept that will serve its purpose.
In literature, it is mostly not distinguished between ‘negative emissions’ and ‘net negative emissions’, and studies that criticize the reliance on future negative emissions tend to question NETs as a whole due to their costs, energy demand, sustainability or immaturity, despite the fact that there are major differences across the wide range of NETs concerning these criteria. Examples are Anderson Peters (2016), Lawrence Schäfer (2019) and New Climate Institute (2019). The title of Anderson Peters (2016) is simply “The trouble with negative emissions”. This could lead to the misunderstanding that negative emissions should not be used at all. The point is, that costs and immaturity do not count as an argument against them: First, it is clear that a fast transition to net zero leads to costs, and second, immaturity is simply a consequence of lack of political will to implement carbon dioxide removal technology. NETs will always remain untested at the required scale, if no one will be obliged to pay for the industrial implementation. EASAC writes in its report (2018): “At present, economic incentives for deploying CCS are inadequate (whether through the very low carbon price or targeted government support), while those for NET development are lacking.”
While the difference between ‘negative emissions` and ‘net negative emissions` (which will always remain crucial, as explained above) is mostly ignored, problems with NETs that would be solvable within a near-term implementation are highlighted.
All in all, this leads to a further delay of the necessary negative emission policies.
Sometimes reports and studies even question the technologies as a whole, but do not question the assumption that these technologies will be used later in the century. An example is Proclim (2018).
The CIEL report (2019), as another example, criticizes technological approaches as a whole (but assumes natural net negative emissions (AFOLU) apart from 2050, according to the IPCC low energy demand scenario P1.)
One of these scenarios depending only on plant-based NETs is described in Grubler et al. (2018). This study concludes that meeting the 1.5°C goal is still possible without non-natural NET deployment, but the scenario of this study includes an afforestation of 300 million hectares and 168 Gt CO2 absorbed by forest sinks between 2020 and 2100. (Comparison: globally 205 Gt Carbon storage potential (758.5 Gt CO2) found by the ETH study Bastin et al. 2019). This might be still quite optimistic, if you consider the total warming effect of forests in snow covered regions, the reduced potential area due to global warming, and the fact that forests are no permanent carbon storages.
Also, critics are concerning the advantages fossil fuel industry tries to get out of NETs: Today, EOR (Enhanced Oil Recovery) is the only industrial use of CO2 that has reached an appreciable scale. (vox 2019) Carbon capture and storage is commercially valuable for oil producers because of carbon dioxide’s usefulness in enhanced oil recovery. (CIEL 2019) It is clear that fossil fuel companies are interested in developing and investing in NETs and also active in the development and promotion of CCS (CIEL 2019), for example through the Global CCS Institute (CIEL 2019).
As a consequence, policies to implement NETs must be constructed in a way that avoids subsidizing the fossil fuel industry, and thus avoids prolonging and expanding a business model that needs to be radically phased down.
One of these policy measures could be a high greenhouse gas levy for any net greenhouse gas emissions. CO2 sequestration from the air (for example through DACCS, direct air carbon capture and sequestration), in return, should be awarded a premium. That premium can be paid from the revenue collected through the CO2-levy.
Actors who would emit CO2, (for example cement plants) but store some of this CO2 permanently, will pay the greenhouse gas levy only for the remaining amount of CO2 emitted. Like this, avoiding emissions would be more attractive than producing them and removing them afterwards.
The levy should at any time exceed the premium paid for sequestration — in order to avoid subsidizing fossil energy use into the future. If the CO2-levy stands much higher than the sequestration premium, CO2 utilization for the production of carbon-based synfuels are incentivized as well. Energy required for the removal must be renewable energy. This could lead to an additional boost for renewables. Realmonte et al. (2019), for example, concludes that deploying DACCS significantly reduces mitigation costs, and that it should be developed and deployed alongside, rather than instead of, other mitigation options.
This should illustrate that possible problems arising from the implementation of NETs, mentioned by CIEL (2018) for example, are solvable, and avoiding (or postponing) NETs does not mean choosing the most cost-efficient way.
Vision
Switzerland exercises its responsibility with determination. In 2021 it starts realizing a just, socially accepted and environmentally compatible way of including negative emissions into its mitigation efforts, in order to stay within its small remaining carbon budget of not more than 265 Mt CO2eq, counterbalances unavoidable non-CO2 greenhouse gas emissions until 2030 and provides other countries its knowledge about including carbon dioxide removal into mitigation policies.
Policy Measures
The goal is to have a socially detrimental pathway for net zero greenhouse gas emissions. Emissions should be prevented from 2030 onwards - or if this is not possible - compensated with NETs.
From a physics point of view, Switzerland’s greenhouse gas emissions do not have to be removed within Switzerland. Crucially, for all negative emissions to be climatologically effective, long term storage has to be achieved. This has to be controlled and guaranteed when deploying negative emissions abroad e.g. by an independent international body. In addition, negative effects on the foreign environment or population have to be ruled out.
Recommendations
Until when do these Policies have to be Implemented?
As seen above, there are several political approaches on how Negative Emission Methods could be implemented. However it is crucial to understand that without political support of these technologies, Switzerland will not be able to stay on the pathways necessary for the Paris Agreement (IPCC 2018). The political approaches must be implemented as quickly as possible.
Distribution of Financial Support in Between the Different NETs
In the first and the third approach mentioned above the state will have to decide how much money will be invested into which negative emission method. At this point we would like to refer to the illustration in the first part of the synthesis which compares the potential, cost and side-effects of each negative emission method for Switzerland.
Since some of the methods still need more research for a clear estimation of their potential in Switzerland it would make sense to create a group of experts which works out a strategy on how to invest into the different technologies. Another possibility would be to create a market for negative emissions and compensate as many emissions as possible with the available money (taking sustainability aspects into account).
Alternatives to Levy on Greenhouse Gases
Taking into account that not all swiss people rely on fossil fuels the same way (for example rural vs urban regions), the money for the financing of NETs could also be raised with a tax independent of the fossil fuel consumption of each individual. This might give financial relief for the people with a high dependency on fossil fuels, but it would not be compatible with the polluter pays principle, which is written down in article 2 of the environmental protection act (USG) and article 74 in the Federal Constitution in Switzerland.
For further proposals on greenhouse gas pricing, see chapter cross-sectoral policies.
Negative Emission Technologies
CO2 removed from the atmosphere can either be stored directly in suitable, secure long-term geological reservoirs, by accelerated natural weathering processes of rock, or converted by plants into biomass, which can then be additionally injected into the soil as a carbon-rich substance.
Carbon Storage
Description of Policy
In Switzerland, saline aquifers should be made accessible to act as storage facilities for CCS projects:
Create a legal framework for the safe geological storage of carbon dioxide, similar to the EU’s CCS directive that has been in place since 2009. In this directive, it is for example written that a site can only be selected if a prior analysis shows that, under the proposed conditions of use, there is no significant risk of leakage or damage to human health or the environment.
The capture of CO2 can be described as noncritical and will probably be regulated by conventional building permit procedures. The Pipeline Act would also be responsible for the legal framework of CO2 transport (Sutter et al. 2013).
Before Switzerland’s saline aquifers are accessible, the effort on the demonstrator of a full CCS chain at KVA Lindt with storage below the North Sea should be scaled up (ETH Zürich, n.d.). Demonstrate the end-to-end feasibility by implementing the 100’000t project in Linth.
- Design of a transport network, for collection of the CO2 within Switzerland and connection to continental storage facilities.
- Make the 32 large point-emitters “capture-ready” through coordination of all ongoing feasibility studies and consistent build-up, sharing and pooling of CO2-specific technical knowledge.
- Develop the necessary regulatory framework (Safety regulations for CO2-transport within Switzerland and cross-border, integration of permanent storage in climate change law, liability and insurance in case of failure of the transport or storage facility, contract with storage company, international agreement between emitting and storing countries).
- Set up the long-term financing (polluter-pay principle, climate fund, compensation project, tax, etc.)
The following laws and acts need to be supplemented by CCS regulations:
- Federal Act on the protection of waters (GSchG, Art. 6-9 und 22-25)
- Waters protection Ordinance (GSchV, Art. 10)
- CO2- law
The technologies DACCS and BECCS (bioenergy carbon capture and storage) require large amounts of inexpensive renewable energy, being more abundant abroad than in Switzerland. To make DACCS and BECCS usable for Switzerland, a legal framework for geological carbon dioxide storage abroad is required.
Misconceptions and misinformation lead to negative perceptions of geological storage in porous rocks, but it has been proven to be safe. For example geological CO2 retention was assessed as 98% over 10,000 years for well-managed reservoirs, and 78% for poorly regulated ones (Alcalde et al. 2018). Natural geological CO2 storage, which has existed for millions of years (Pearce et al. 2004), and anthropogenic analogs (seasonal natural gas geological storage) have additionally demonstrated long term safety.
A specific example for carbon storage abroad are the northern European offshore storage projects (either CO2 transport or local capture via DACCS). For example, Norway is considering to open up their offshore geological reservoirs to all European CO2 emitters by 2024. The Norwegian Parliament will make an investment decision for the project in 2020/2021. The project will then be able to commence operations in 2023/2024 with a planned capacity of approximately 5 million tons of CO2 per year (Eckle et al., 2019).
Description of Technology
Highly concentrated CO2 from the flue gas or from ambient air is extracted and pumped to underground rock formations, capable of providing long term geological storage. The CO2 is thereby first trapped in pores of porous rock, such as sandstone. In a second step, it is dissolved into the already present brine, forming carbonic acid within the brine. The brine reacts in a third step with minerals to form carbonates. A suitable underground geological formation is also equipped with an impermeable cap rock to provide redundancy. An analogue technology has been used for seasonal natural gas storage in Europe and the US for more than 45 years. In the US alone 3720 billion cubic feet of natural gas are stored between seasons (eia 2020). The volume is equivalent to 229 Mt of CO2. The seasonally stored volume of natural gas in Germany is equivalent to 61 Mt of CO2.
Globally, CO2 can be stored in different geological formations (Fuss et al. 2018):
- Empty oil and gas wells. These were gas-tight for millions of years and thus enabled the formation and storage of these fossil hydrocarbons.
- Coal seams that have not been mined: Carbon can store CO2 like "activated carbon".
- Basalt rock: young basalt rock reacts with CO2 to form carbonate rock. CO2 is thus securely bound (Snæbjörnsdóttir et al. 2014)
- Saline aquifers: deep, water-bearing rock strata - these could probably absorb 500 Mt CO2 in Switzerland (see subsection impact below), worldwide the potential is many times bigger (IPCC 2018).
Impact
According to the lPCC SR 1.5 report, there are huge storage capacities of at least 2,000 Gt and a maximum of 55,000 Gt CO2 available worldwide (for comparison: currently less than 40 Gt CO2 are emitted worldwide per year). The security of the storage facilities is considered high in IPCC SR 1.5. Thus, worse CO2 storage facilities should retain 78% of the CO2 over 10,000 years, whereas good CO2 storage facilities should retain 98%.
The bottleneck is not the storage option, but the separation of CO2 from the air or exhaust gases. Upon contact with Prof. Dr. Larryn Diamond, a leading Swiss geologist in the field of carbon storage, the potential storage in Switzerland is in the order of 500 Mt CO2. There is an opportunity to investigate the suitability of Switzerland’s subsurface for CO2 storage in conjunction with the current subsidy programs intended to characterize the subsurface for geothermal energy utilization (Eckle et al. 2019).
Financing
The storage of CO2 in underground rock formations is the same for the capturing methods DACCS, BECCS and CCS. For information about the prices see the regarding subchapters.
Carbon Usage
Description of Technology
Instead of storing the CO2 in a safe deposit, it could be used as a raw material. The demand for CO2 itself is extremely low compared to the emissions. It is suitable as a refrigerant, as carbon dioxide for beverages, for fire extinguishers, etc. A much higher demand results if the CO2 is converted into other substances with a high energy input. In this way, CO2-neutral fuels "Synfuels", plastics and chemicals for the chemical industry can be produced. In principle, this requires reversing the combustion of the carbon with the help of renewable energies - the CO2 must be reduced again. This is done in reactors, where the CO2 is reacted with regeneratively produced hydrogen and suitable catalysts.
It is important to note that the use of CO2 does not contribute to negative emissions, as the substances produced from it are re-combusted relatively quickly, thus releasing the CO2 back into the atmosphere.
Impact
The impact is expected to be small. If it is possible to safely store CO2 in suitable geological formations as predicted in the studies, there is little reason to believe that CO2 removed from exhaust gases or air will be converted back into synfuels or chemicals with a high energy input instead of being disposed of directly. Calculations by Gabrielle et al. (2020) and Hostettler (2020) have shown that the demand for renewable energies for the production of synfuels from CO2 is five to seven times higher (Hostettler 2019) than if kerosene is burned conventionally and then neutralized by DACCS. Gabrielle et al. (2020) arrive at comparable results for the question of whether raw materials for the chemical industry should be produced using CCUs (Carbon capture and usage).
Financing
Due to the enormously high energy demand, this technology will have a very difficult economic position compared to the further use of oil as a raw material in combination with DACCS or CCS for CO2 extraction. As the global demand for crude oil will collapse due to climate protection, the prices for crude oil for non-substitutable applications (plastics, aviation, chemical industry, etc.) will fall sharply, as crude oil will only be extracted from the most suitable sources - even in combination with DACCS or CCS low costs will result, while CCU and DACCU are very costly, especially due to the downstream conversion of CO2.
Carbon Capturing (DACC)
Description of Policy
It must be stipulated by law that DACCS installations financed by Switzerland abroad are credited to Switzerland as negative emissions. This is possible because of the Paris Agreement 2015 Article 6.2, 6.3, 6.4 (Christoph Beuttler Jens Leifeld et. al 2019).
There are many indications that DACCS is an interesting and important option for Switzerland, but that the procedure itself will not be carried out on Swiss soil. The main arguments against this are the large demand for renewable energies and the fact that it makes sense to carry out DAC only in places where the CO2 can be stored immediately. Only in this way high transport costs can be avoided.
Description of Technology
Direct Air Carbon Capture and Storage (DACCS) means that CO2 is extracted directly from the air. The systems suck in normal air and bring it into contact with a sorbent that reacts selectively with CO2, thereby absorbing it. Once the CO2 absorption capacity of the sorbent is reached, it is heated to release the CO2 as a pure substance. The sorbent can then be used again for CO2 removal.
The sorbent can either be a granulate that binds CO2 to itself on the surface or a liquid that can absorb CO2 in large quantities. Suitable granules are amine-functionalized substances (climeworks, n.d.) while the liquid used in many chemical processes is sodium hydroxide solution.
Since CO2 is present in the atmosphere with a concentration of only 0.04 %, the energy requirement for "filtering out" the CO2 with approx. 250 kWh of electrical energy and approx. 1800 kWh of thermal energy per ton of CO2 is relatively high (Fasihi, Efimova, and Breyer 2019). Most of the energy is required as thermal energy for heating the sorbent so that it can release the CO2. A smaller part is required in the form of electrical energy, mainly for the operation of the fans.

Impact
The IPCC SR 1.5 Report refers in chapter 4.3.7.5 on Carbon Dioxide Removal mainly to the study by Fuss et al., (2018) According to this study, DACCS will be able to remove 0.5-5 Gt CO2 per year from the air at a cost of 100-300 US$/t CO2.
In Switzerland itself, the potential of DACCS will be very small: no very cheap renewable energies are available. However, if plants built by Switzerland abroad are allowed to remove CO2 from the atmosphere and this CO2 is credited to Switzerland, the technology has enormous potential.
The limit is thus set by the cost: the cost of DACCS will make it more attractive for most applications to avoid CO2 emissions instead of having the CO2 subsequently removed from the air by DACCS. From a global perspective, the storage capacities for CO2 extracted from the air are sufficiently large: minimum 2,000 Gt CO2, maximum 55,000 Gt CO2 (IPCC 2018).
Financing
Although the technology has been researched, no major facilities are yet in operation, so the current price is between 600-800 Swiss francs per ton of captured and sequestered CO2. It is assumed that the price will decrease to about 100-300 US$ per ton (Fasihi, Efimova, and Breyer 2019) as soon as the technology is used on a larger scale.
Social Compatibility
If it should be necessary to use NETs abroad, negative effects on the foreign environment or population have to be ruled out.
Questions and Uncertainties
As long as it is free to dispose of CO2 in the atmosphere, DACCS cannot work at all - there is no market for CO2 removal from the air. Even today's existing CO2 control levies are far from the current price of DACCS, which is currently in the range of 600 - 800 Swiss francs per ton of CO2. By contrast, future CO2 levies are likely to be higher than the likely price of DACCS in the future (approx. 100-300 Fr./t), so the method in itself has enormous future potential. The open question remains as to who will finance the scaling up of this technology and thus help to reduce costs while it is still more expensive than the CO2 taxes. This hurdle must be overcome in order to make the breakthrough possible.
Carbon Capturing (Bioenergy Carbon Capture)
Description of Technology
Bio-energy with carbon capture and storage (BECCS) is a negative emission technology to extract CO2 from the carbon cycle (atmosphere, lakes or ocean) and store it securely in geological underground formations for millennia. It is a negative emission technology highly advocated by the IPCC Special Report on Global Warming of 1.5 °C because of its renewable energy allocation, low cost, low ecological footprint and long-term storage capability. However, the high land use (scientifically justified), loss of biodiversity (scientifically justified), and safety concerns of geological storage (scientifically unsupported) are critics of the technology.
Fast growing biomass, e.g. algae, grass, bushes and trees are grown, thereby locking ambient CO2. The heat during the subsequent incineration process can be used to generate electric power or provide heat for the industry or to feed a district heat network. Highly concentrated CO2 from the flue gas is extracted and pumped to underground rock formations, capable of providing long term geological storage. The CO2 is thereby first trapped in pores of porous rock, such as sandstone. In a second step, it is dissolved into the already present brine, forming carbonic acid within the brine. The brine reacts in a third step with minerals to form carbonates. A suitable underground geological formation is also equipped with an impermeable cap rock to provide redundancy. An analogue technology has been used for seasonal natural gas storage in Europe and the US for more than 45 years. In the US alone 3720 billion cubic feet of natural gas are stored between seasons (eia 2020). The volume is equivalent to 229 Mt of CO2. The seasonally stored volume of natural gas in Germany is equivalent to 61 Mt of CO2.
Compared to afforestation, BECCS needs 2.5 times less land use to sequester the same amount of CO2. However, direct air capture and storage (DACCS) needs an additional 750 times less land use (including PV to power DACCS)(climeworks n.d.) and is therefore the preferable technology compared to BECCS, despite the higher price at this point in time. Besides, water usage is close to non-existing in DACCS.
Impact
The predicted future potential for BECCS is sufficient to remove a substantial part of Switzerland’s emissions. The two main challenges for large-scale implementation are biomass production and further research in identifying suitable geological storage locations. Biomass production is in close competition with food production, in Switzerland and globally. Biomass as a waste product of food production can be utilized for BECCS. Dedicated analysis for Switzerland is non-existing at this time. The Biomass of crop residues captures approximately 1.4 Mt CO2/year (see section biochar). The IEA Greenhouse Gas Technology Collaboration Program (IEAGHG) assessed the global potential for BECCS to 10 Gt CO2 per year (IEAGHG 2019).
Financing
No pricing estimations exist so far for Switzerland. Global assessments estimate 100-200 $/t CO2 (Fuss et al. 2018).
Social Compatibility
Unjustified fear of geological CO2 storage is evident amongst Swiss citizens. False friends, e.g. fracking, geological heat or nuclear waste storage produce negative affections towards the technology. In addition, the immense land use footprint is competing with food production and lowers biodiversity, if not well controlled and optimized for CO2 sequestration.
Carbon Capture in Industry
Description of Technology
CCS (carbon capture and storage) is the process of capturing waste CO2 usually from large point sources, transporting it to an underground facility and storing it permanently. Due to higher CO2 concentrations at point sources than in ambient air, the capturing process is more energy efficient at point sources.
In Switzerland there are currently about 32 big point sources (cement industry, waste incinerator plants, refineries, 1 gas power plant, chemical industry and biomass plants). Together, they emit about 5 million tons of fossil CO2, plus 2 million tons of biogenic CO2 (ETH Zürich, n.d.).

The following reasons indicate that CCS appears to be the most promising path to drastically reduce CO2 emissions in swiss industry (ETH Zürich, n.d.):
- From a climate protection perspective, taking action to decarbonize large emitters is a must. Capturing CO2 and storing it in deep geological formations is much better than continuing CO2 release in the atmosphere
- From a physical perspective, the mechanism governing storage and long-term stability are well understood. CO2 will be trapped in microscopic rock pores, the same mechanism that has trapped natural gas for millions of years.
- From a technical point of view, storing more than 23 Mt of CO2 over the course of 20 years at Sleipner and other sites in Norway show that CCS works.
- From a safety point of view, the long-term stability of the storage can be monitored. It is possible to measure where the CO2 is and that it stays there. In the Northern Lights project, extensive testing of the measurement equipment is currently underway in preparation of opening the first storage site (Aurora).
- From a legal point of view, the EU has already set the regulatory framework in 2009 with the Directive on Geological Storage of Carbon Dioxide (EU CCS Directive). The Norwegian Aurora site will comply with the EU CCS Directive. Further examples of CCS-Regulations can also be found outside Europe (California).
The chemical industry for example faces a special challenge in being desilicated, as an overwhelmingly large fraction of chemical products contains carbon, mostly originating from fossil-C. Gabrielle et al. (2020) compared three technology chains (CCU route, Bio-route and CCS route) that enable a carbon neutral chemical industry, and highlighted the following advantages of the CCS route:
- It can exploit the existing technology and the infrastructure in place of the current petrochemical and chemical industry, without the need of a complete reshaping of it.
- In the case of methanol production, the CCU route results in an electricity consumption 10 to 25 times higher than that of the CCS and BIO routes (excluding the electricity required for heat production), mostly due to the electricity required to produce hydrogen, and the Bio route requires a land capacity about 40 and 400 times higher than that required by the CCU and CCS routes, respectively.
- CO2 capture from point sources and/or from air plus permanent CO2 storage in geological formations constitute the key elements of the negative emissions technologies, on which future scenarios targeting a global warming below 1.5 ° or 2 °C rely.
If applied to flue gas from fossil based materials or cement production, CCS is at best carbon neutral. It only produces negative emissions when carbon dioxide from biogenic material combustion is captured (which is denoted as BECCS, see 3.2.2). Nevertheless, it plays a major role in decarbonizing the industry sector in the context of 1.5 °C and 2 °C pathways, especially in industries with higher process emissions, such as cement, iron and steel industries. IPCC (2018) states: “In the IPCC 1.5 °C-overshoot pathways, CCS in industry reaches globally 3 GtCO2 yr−1 by 2050, albeit with strong variations across pathways. Given the projected long-lead times and need for technological innovation, early scale-up of industry-sector CCS is essential to achieving the stringent temperature target. Development and demonstration of such projects has been slow, however. Currently, only two large-scale industrial CCS projects outside of oil and gas processing are in operation (Global CCS Institute, 2016).”
Volkart et al. (2013) found that the implementation of CCS leads to life cycle GHG emission reductions of 68–92% for fossil power generation and 39–78% for cement production whilst to negative ones for wood power generation.
CCS was already discussed in the context of the need for up to seven natural gas combined cycle (NGCC) power plants after the decision to phase out nuclear energy (Sutter et al. 2013), (Wallquist and Mischa Werner, n.d.), (Thalmann and Vielle 2019).
Broadly speaking, there are three different CO2 capturing methods:
- pre-combustion capture (fossil fuel is decarbonized before the combustion) (no installation into existing plants possible)
- post-combustion capture (installation into existing plants possible)
- oxyfuel combustion capture (nitrogen is isolated from the process, which leads to a pure CO2 waste gas stream)
CCS should be applied in the following two industrial processes in Switzerland:
Cement sector
There are 5 cement plants in Switzerland, each of which emits between 500’000 and 600’000 t CO2 per year, together 2.7 MtCO2 per year, which is 38% of the CO2 rich flue gases in Switzerland (Kober et al. 2019)
These emissions occur from different processes:
“Emissions of geogenic CO2 occur during the production of clinker, which is an intermediate component in the cement manufacturing process. During the production of clinker, limestone, which is mainly calcium carbonate (CaCO3), is heated (calcined) to produce lime (CaO) and CO2 as by-product. The CaO reacts subsequently with minerals in the raw materials and yields clinker. During this reaction step no further CO2 is emitted. Clinker is then mixed with other components such as gypsum to make cement. Blasting operations in the limestone quarries are another source of emissions for both CO2 and precursor greenhouse gases such as NOx, CO, NMVOC and SO2.” (Switzerland’s Greenhouse Gas Inventory).
Total annual geogenic CO2 emissions: about 2 Mt CO2 (Chapter Industry), total CO2 emissions from fossil fuel combustion: 378000 t CO2 in 2018.
An additional amount of the cement plant’s CO2 emissions occurs from fossil fuel combustion. If the total annual emissions from cement industry are assumed to be 2.7 million t CO2 (Kober et al. 2019), using the values of the life cycle analysis by Volkart et al. (2013), cement plants could reduce their CO2 emissions by between 1 and 2.1 million t CO2. Between 0.6 million and 1.7 million tons CO2 would remain (under current production rates) and would have to be counterbalanced through other negative emissions methods, if the moratorium on new infrastructure demanded in chapter cross-sectoral policies is not implemented.
Waste incinerator plants
There are 30 waste incinerator plants in Switzerland (ETH Zürich, n.d.). Together, they emit a total of approximately 4.4 million tons of CO2 per year - about half of these emissions are fossil-based, and the other half biogenic (ETH Zürich, n.d.). While Carbon Capture has been done for decades (for example in the chemical industry at Lonza, ammonia-based), the application to waste-to-energy plants is starting now. One waste-to-energy plant in the Netherlands uses CCS since 2019 (Duiven, NL). At KVA Lindt, there is currently a demonstrator for the full CCS chain in preparation.
Amine-based CO2 capture can be implemented at scale at Waste-to-Energy-plants with a CO2 capture efficiency of about 90% (vbsa 2019). A recent IEAGHG study suggests that increasing CO2 capture rate to 99% could be achieved at an 8% cost increase. Thus, more than 90% of the biogenic 2.1 Mt CO2 could be counted as BECCS, and thus negative emissions.
As CO2 capture requires substantial amounts of thermal energy and this thermal energy could be converted to electricity, using it for CO2 capture has an opportunity cost for power generation of approximately CHF 46 per ton of CO2 captured, rising to CHF 75 if the energy-intensive steps of purification and liquefaction are included.
Although VBSA/ASED/ASID expects the waste composition to shift toward less plastic waste and more biogenic waste, the overall CO2 emissions from WtE-plants (waste-to-energy plants) will most likely rise, and decarbonization of the waste sector will need to come from capturing CO2 emissions at WtE plants, as the total quantity of waste for incineration is not expected to go down materially until 2050 (Eckle et al. 2019).
For the following industrial processes, feasibility of CCS should be verified:
If realized: natural gas combined cycle (NGCC) power plants (approx. 1.3 Mt CO2 per year), existing power plants: Axpo Tegra AG Domat/Ems, Monthel AG Monthey
Chemical industry (Lonza AG Visp)
For example ammonia production, carbide production, ethylene production, niacin production. Note: Processes releasing other greenhouse gases, such as N2O, must be addressed by methods beyond CCS.
Impact
Regarding the life cycle analyses (Volkart, Bauer, and Boulet 2013) and the numbers of capturing efficiency (Eckle et al. 2019), CCS can contribute significantly to decarbonize Switzerland’s industry, plus provide a negative emissions potential of at least 2 million tons CO2 through waste incinerator plants.
Financing
CCS in waste incinerator plants:
“Current very rough estimates indicate the cost for CO2 capture, transport, injection and monitoring costs of around CHF 340 per ton of CO2 with the expectation to go down to CHF 110 per ton of CO2 in the next 10 years (amounting to CHF 1.3 and 0.4 billion, respectively, in annual costs for all Swiss WtE CO2)” (Eckle et al. 2019).
CCS elsewhere:
The estimated current cost of CO2 avoided (in USD2015) ranges from $20–27 tCO2−1 for gas processing and bio- ethanol production, and $60–138 tCO2−1 for fossil fuel-fired power generation up to $104–188 tCO2−1 for cement production (Irlam, 2017) (IPCC 2018).
Social Compatibility
Identified key hurdles include the availability, accessibility, and acceptance of CO2 storage sites, but not their safety, which has been extensively proven (Kissinger, Herold, and De Sy 2012).
Questions and Uncertainties
The capture technology requires additional energy resulting in higher energy (electric or fuel) consumption. This could be compensated by a high CO2 price to make this economically feasible.
Enhanced Weathering
Description of Policy
For the implementation of Enhanced Weathering on a large scale in Switzerland, the legal framework is sufficient, while financial incentives are required to make its application rewarding for landowners.
Description of Technology
Weathering is a slow natural process removing a fraction of the CO2 from the atmosphere by the formation of carbonate mineral rocks. It has been the main CO2 sink over the past 850 millennia removing 1.2-1.9 ppm over 1000 years (Barnola et al., 2003). CO2 forms carbonic acid with water, e.g. in raindrops, which react with alkaline rocks such as calcite (CaCO3, limestone or chalk) or forsterite (Mg2SiO4). Calcite dissolved by H2CO3 (carbonic acid) will form Ca(HCO3)2 which can only exist in a solution. All CO2 absorbed into ocean water through the addition of ground calcite will slowly be converted into carbonate minerals by calcifying organisms.
For enhanced weathering or enhanced silicate rock weathering, favorable minerals are slashed and ground into a fine powder. The powder is then mixed with soil in agriculture, thereby also fertilizing the soil with Calcium, Magnesium, and Silicon. A positive effect on crop yield is to be expected. Alkaline rock powders (calcite etc.) are only applied to agricultural soils with lower-than-desired pH (pH<5.5). The reaction of calcite with acidic soil leads to calcium bicarbonate, which is soluble. As mentioned before, dissolved carbonates are prone to outgassing, especially in agricultural soils, where stronger acids (nitric acid, sulfuric acid) are present in larger amounts (plant nutrients) and are able to displace bicarbonates. On a global scale, enhanced weathering offers also the potential to reduce ocean acidification. If the milled powder is directly added to the ocean, the technique is referred to as ocean alkalization.
Another approach is to recycle finely ground cement rather than calcite or forsterite. During the cement production calcite is processed to slaked lime in a kiln. The calcite thereby releases CO2 which the slaked lime partially takes up again during the curing process of the cement. Milled and dispersed cement still contains slaked lime which takes up carbonic acid much more efficiently and results in a net CO2 take up if the CO2 released while processing the calcite in the kiln is captured.
Impact
Beuttler et al. (2019) estimates 2.5Mt CO2/year (from recycled cement, which also requires a sustainable CO2 source, e.g. DAC). Beerling et al. (2020) calculated potentials for Germany (77 Mt CO2/y), Italy (43 Mt CO2/y) and France (104 Mt CO2/y). For Switzerland 3.4-4.0 Mt CO2/y are estimated if the previously stated values are scaled from each countries to Switzerland’s cropland area.
To remove the annual direct (indirect) Swiss CO2 emissions of 44 (112) Mt CO2 a total of 100 (255) Mt of pure calcite or 35 (89) Mt forsterite is needed. Calcite is abundant in Switzerland’s Jurasian mountains.
Financing
160-190 CHF/t CO2 Cost adopted from calculations for Germany (Beerling et al. 2020)
20 - 1000 CHF/t CO2 (Fuss et al. 2018).
Social Compatibility
Enhanced weathering is reducing the acidification of the oceans (likely) and increasing crop yield (likely). The risks and indirect consequences as result of locally increased alkalinity are uncertain. Acceptance with farmers and landowners in using enhanced weathering has to be developed, as soon as enhanced weathering is proven to be safe.
Afforestation, Reforestation and Wood Usage
Description of Policy
Create the legal framework to tap the full potential of CO2 storage through reforestation and afforestation and wood usage in Switzerland, without compromising biodiversity.
Description of Technology
This section covers wood-related negative emission technologies. On one hand forest policies are discussed. They include active afforestation, natural reforestation and the management of existing forests. On the other hand, climate-friendly options for the wood usage are described.
Afforestation is the active establishment of a forest or stand of trees in an area where there was no previous tree cover for the last 50 years or more. In contrast, reforestation is a natural process in areas covered by trees in the past. An improved forest management is the third pillar of forest policies.
From a global point of view, photosynthetic carbon capture by trees is likely to be among one of the most effective global strategies to limit the rise of CO2 concentrations. Consequently, a number of international initiatives [such as the Bonn Challenge, the related AFR100, and the New York Declaration on Forests have established ambitious targets to promote forest conservation, afforestation and restoration at a global scale. The latest special report by the Intergovernmental Panel on Climate Change (IPCC) suggests that an increase of 1 billion ha of forest will be necessary to limit global warming to 1.5 °C by 2050.
A climate-friendly usage of wood provides possibilities of energy and material substitution and additional mid-term CO2 sequestration. A certain ratio of harvested wood can be used in long-lived wood products, mainly in construction and furniture, and thereby store CO2 during their lifetime.
Furthermore, wood used as construction material can substitute energy intensive materials (concrete and steel). This substitution effect is the most important in the long-term and might reduce the CO2 emissions significantly related to cement production. At the end of its lifetime, the wood products - together with wood explicitly harvested for this purpose - can be used for energy production where it substitutes fossil energy sources. The combination of wood combustion with CCS technologies as BECCS provides authentic negative emission potential beyond the substitution potential.
In 2011 the Swiss government defined the strategic direction of the forest policy (Hilaire et al. 2019). The second of the 11 main objectives is the mitigation of climate change by dedicated forest policy and wood usage while maintaining resilient forests under changed climate conditions. The strongly related “Wood Action Plan” aims at a sustainable wood production and cascaded use of wood including climate-friendly construction (IPCC 2018).
Impact
Since 1990 CO2 uptake by afforestation in Switzerland has been small (about 20 kt CO2 eq. per year) and outweighed by deforestation by more than seven times. The afforestation area is very small (about 0.05 kha per year compared to forest management area of 1’250 kha) and consequently, the potential for carbon dioxide removal is very small. In contrast, the potential to store CO2 through natural reforestation is about 730 kt CO2 per year. However, abandoned areas are decreasing. The potential for carbon dioxide removal by forest management is significantly larger. During the last three decades, the Swiss forest sink provided between 1.6 million t CO2 eq. per year (2014) and 4.6 million t CO2 eq. per year (1995) in CO2 storage, with the exception of year 2000 when the forest constituted a source of 4.2 million t CO2 eq. per year after the catastrophic storm “Lothar”(FOEN 2019e).
Different wood management strategies can lead to very different short- and long-term effects (Hofer et al. 2007). A strategy of reduced forest maintenance is only efficient in the first decades before the forest turns into a CO2 source. Forests that are not maintained properly have a higher risk to become a massive source of CO2 in case of natural disasters, like storms or wildfires. Therefore, forest resilience is an important objective for climate policy. In general, the CO2 sinks in natural and civilization wood cycles are limited and will be exhausted somewhen between 2050 and 2100 depending on the wood strategy.
The Swiss domestic wood harvest is rather constant with 5.2 million m3/year in 2018. But it could be sustainably increased to 9 million m3/year (Fischlin et al. 2006). Apart from increasing wood production, increased import of wood can be considered, but poses danger of “Land Grabbing” or deforestation abroad. In 2018, Switzerland used 11.2 million m3 of wood, thereof 54% for energy production, 24% for massive wood products, 19% for paper and cardboard (BAFU 2019). Swiss buildings currently store 45 Mt CO2 eq. with around 15% of all Swiss buildings being wood constructions (Starck 2016). In 2018, 14.2% of all new constructions were wood based (BAFU 2019). Only 4.2% of the Swiss primary energy consumption is provided by wood. Therefore, there is still a big potential for enhancing the wood usage in Switzerland.
Material substitution with domestic wood alone could provide a CO2 long-term reduction of -1.6 Mt CO2 eq. per year, energy substitution could provide -4.0 Mt CO2 eq. per year (Hofer et al. 2007). As the CO2 is likely to be re-emitted, these strategies are carbon neutral at best. True negative emissions can be created by applying CCS techniques in the wood combustion. This might offer another potential CO2 reduction close to the CO2 saving by energy substitution.
Hence, the wood management strategy should combine different measures. Primarily maximizing the sustainable production of wood and long-lived wood products. Secondly, to maximize the use of raw wood and wood products (cascaded use) as an energy source whereby CCS techniques should be applied.
Compared to other negative emission technologies the wood related techniques are broadly known. The technology is ready for large scale implementation (Fuss et al. 2018). Forest management strategies with focus on reducing CO2 emissions are already being implemented by forest corporations in order to sell CO2 certificates on the National voluntary market (Christoph Beuttler Jens Leifeld, Martin Schmid et al. 2019) The proposed strategy is ready to be implemented and could be stimulated by known measures.
The potential of carbon storage by growing forest masses is limited and could be exhausted in a few decades. But strategic use of wood products offers additional CO2 reduction and increases the time spans for active CO2 removals. CO2 can be stored in wood used as long living construction materials. More importantly, wood can substitute CO2 intensive materials such as concrete and steel.
Financing
The estimated costs per saved ton of CO2 eq. for afforestation / reforestation range between 1 and 100$ globally (Fuss et al. 2018) A more narrow estimate is reported between 5 and 50 USD (Fuss et al. 2018).
Social Compatibility and Risks
Afforestation and reforestation reduce the area available for agriculture. Food security might therefore be an issue, in particular if Switzerland does not want to rely on foreign food supply. Also, biodiversity might be affected negatively because afforestation and reforestation might reduce the abundance of traditional cultivated land ecosystems.
The emission of particulate matter is expected to increase if more wood is used as an energy source, in particular if burned in private houses, which may have health consequences that need to be taken into account.
The CO2 uptake of forests and bushes decreases with time and reaches near zero after typically 100-200 years when the forest becomes mature. To maintain a high level of CO2 uptake, forests have to be well-managed by removing old trees and shrubs. Forests have a lower albedo than open land (Schwaab et al. 2015) meaning less sunlight is reflected back to space and creates additional heat. This reduces the mitigation effect in particular in mountainous areas (Schwaab et al. 2015). However, in Switzerland the total effect of additional forest is always positive in terms of negative emissions.
As the potential of afforestation in Switzerland is limited, afforestation abroad might be a targeted strategy. Additional substantial risks are “Land Grabbing” or afforestation on agricultural land, which is currently much needed to provide food for the local population.
Afforestation cannot be considered as a reliable permanent NET, due to possible loss of stored carbon because of storms, fires etc. Afforestation should only serve as an additional method not counting as a NET. Biochar or BECCS with biomass from afforestation on the other hand provides this reliability.
Biochar
Description of Policy
Residual biomass from agriculture and forestry can be transformed to biochar by pyrolysis (“charring”). The biochar is then mixed with soil to allow long term storage. The mean residence time of biochar in Swiss soils must be evaluated and controlled in order to evaluate the efficiency of biochar in Switzerland as a NET.
Description of Technology
Residual biomass from agriculture (various types of straw/stover, preferentially low in nitrogen) and forestry (bark, sawdust, branches) are dried or harvested in a dry state, compressed if necessary and heated in an inert atmosphere to 500-600°C. At this temperature, biomass releases a mixture of flammable gases and is chemically transformed into a chemically profoundly altered material: charcoal. Once ignited, the process releases around 50 % of the caloric value of the dry biomass. This translates to heat energy in the order of 2.1-2.8 MWh per ton of biomass. For every ton of dry biomass, between 200 kg and 250 kg C is sequestered in a stable form (IUCN, n.d.), provided the pyrolysis conditions were as mentioned before. This translates to 732-915 kg sequestered CO2 per ton of pyrolyzed biomass. The removal and pyrolysis of agricultural residue from fields as well as the application of the resulting biochar have a number of beneficial agronomic effects, such as:
- removal of plant pathogens and insect pests
- reduction in competition for plant nutrients between soil microorganisms and plants (Bastin et al. 2019)
- fast and virtually limitless increase in soil carbon content
- improvement in soil water holding capacity, conductivity and infiltration
- reduction of CH4 and N2O emissions in certain soils (IPCC 2018)
Technical challenges in the implementation of wide scale biochar generation are:
- elevated moisture content at harvest of some agricultural residues
- lack of pyrolytic burners able to generate biochar under optimal conditions
- lack of efficient heat utilization systems
- need for decentralization of biochar production to minimize transport of very lightweight feedstock
- Logistics of biochar: conventional combustion of biomass leaves little solid residue whereas large amounts of biochar needs dedicated transport of goods.
- Permanence of biochar, stored in agricultural soils: depending on soil type and pyrolysis method between a few decades and several centuries (FOEN 2019d) and (IPCC 2018) - mean residence times lie between 44-610 years.
- Permanence of biochar stored in clay (anaerobic conditions in a final repository): stable conditions for millennials (similar to lignite / hard coal)
Impact
There is no data for agricultural residue production in Switzerland, but it is safe to assume that in cereals, biomass is allocated at least 50% to the straw fraction (UN 2014) Knowing that the cereal yield was 0.88Mt in 2018 (Griscom et al. 2017), straw biomass was at least 0.88Mt.
Although there is no clear data on forestry waste generation, Gregg and Smith (2010) estimate that forestry residue is slightly more abundant than cereal straw in Switzerland. Assuming 0.88Mt cereal straw and 1Mt forestry residue, around 1.4Mt CO2 could be sequestered annually using the biochar technology.
Financing
Currently, a commercially available, shipping container sized pyrolysis unit (PYREG) can process 750 t dry biomass per year (equivalent to straw from 100-150 hectares of cereal cultivation), resulting in 150-187 t of sequestered C annually. The purchase price of the unit is CHF 400’000 and it can generate at least 1500 MWh/yr of heat energy. When calculated with a heat energy price of CHF 0.07 per kWh, the unit can generate around CHF 100’000 worth of energy per year. Loose cereal straw is worth CHF 60 per ton resulting in feedstock costs of CHF 45’000 per pyrolysis unit. Economical payback is therefore reached in less than 10 years, excluding the value of biochar and related carbon credits. For maximum economic feasibility, it is important to design the pyrolysis unit according to the heat energy needs, not feedstock availability.
Social Compatibility
As with all dry biomass/fuel handling, precautions need to be taken to minimize the risk of dust explosions. Unlike other combustion technologies, pyrolysis creates large amounts of solid combustion residues (biochar) that need to be handled. This can be a logistical challenge in densely populated areas.
Questions and Uncertainties
Of all vegetation types found in Switzerland, only grasslands do not produce a harvestable residue suitable for conversion into biochar. Grasslands, however, constitute a large proportion of land area in Switzerland. Fall harvested crops (grain corn, sunflowers, soy beans) typically have elevated residual moisture, requiring some kind of drying in order to be pyrolyzed. Open air passive drying techniques need to be developed for maximum energy efficiency. The PYREG pyrolysis unit produces 210-280 kW heat power, suitable for heating between 50 and 100 households. Smaller pyrolytic heating systems are required for large scale adoption of this technology. Biochar pick up and distribution on the agricultural land needs to be well organized. As mentioned above the mean residence time of biochar has not been determined for Swiss soil yet.
Soil Carbon Content/ Sequestration
Description of Policy
The technological readiness of soil carbon sequestration (SCS) is high. Thus, implementation is mainly hampered by difficulties in political coordination, monitoring and appropriate attribution of cost.
- Establishment of a comprehensive SCS modeling and monitoring framework in Switzerland with the potential to scale to other countries and contexts.
- Set up a funding structure for SCS in Switzerland alongside a broad information campaign on how to take up SCS for potential practitioners.
- Embed SCS into Swiss NETs policy framework, with appropriate consideration of the benefits and risks of SCS in the Swiss NET portfolio.
Description of Technology
Soil carbon sequestration (SCS) denotes methods of land management that increase the soil organic carbon content in a manner that leads to a net removal of CO2 from the atmosphere (Griscom et al. 2017). How much carbon is retained in the soil depends on the balance of carbon inputs into the soil (e.g. from litter, residues, roots, manure) and carbon losses from the soil (mostly through respiration, increased by soil disturbance) (Griscom et al. 2017). Thus, practices that either increase inputs, or reduce losses or both, can promote SCS (Griscom et al. 2017). In short, SCS is a collection of land use management techniques that tip the balance of carbon inputs vs outputs in favor of building up carbon in soils.
More concretely, practices that are estimated to add net carbon to the soil include: Use of cover crops, leaving harvest residues on the field, return of organic residues on the field via fertilization (here the fertilizers are farmyard manure, slurry, compost), planting deep rooting crops, grass clover leys in crops rotations, agroforestry, diversified crop rotations, deep ploughing and no-tillage practices (Lewis et al. 2019).
Impact
Current carbon stock changes in soil are estimated by the Swiss Soil Monitoring Network. The component that most contributes to carbon losses or gains estimated by FOEN is termed “net carbon stock changes in soils due to use of mineral and organic soils due to land use changes”, which may be interpreted as the rate of SCS in Switzerland resulting from the few currently utilized land use management techniques (which are not optimized for SCS). Switzerland has 4100 kha of mineral soil and 27 kha of organic soil. The rate of SCS in Switzerland for the last two decades has been negligible.
To our knowledge, only one report has provided an estimate for the potential - that is, what might be sequestered by instead of what is sequestered - of SCS technology in Switzerland. Beuttler et al. estimate that the combined potential for SCS in Switzerland from both agricultural (0.7 Mio t CO2 per year) and soil (1.9 Mio t CO2 per year) (Lewis et al. 2019) sequestration is 2.6 Mio t CO2 per year.
One French study estimated the potential for SCS on agricultural land is 0.63 ton carbon per hectare per year (UN 2014). Extrapolation of this potential to the entire Swiss cropland gives a potential of 925 kt CO2 per year. Swiss long term experiments for SCS on grassland yield a SCS potential of 0.28 t C per hectare per year (Bastin et al. 2019). This sums up to a total Swiss grassland potential of 945 kt CO2 per year. Taken together, these two give a potential of 1.87 Mio t CO2 per year (Lewis et al. 2019). This corresponds to about 4-5% of total production-based Swiss CO2 emissions. These estimates lie in between the minimum 0.03 t C per year and maximum 1 t C per hectare per year given by Smith et al. for cropland and grassland on a global average (FOEN 2019d).
A different approach, deep ploughing, may provide additional SCS potential. Studies from Germany (Thürig and Traub 2015) and New Zealand show that the shift of non-easily decomposable carbon into greater depths of the soil, where it is sequestered due to longer residence times, yields gains in carbon sequestration. Beuttler et al. (2019) estimate that applying this deep soiling technique on 5000 ha on an annual basis could offer a potential of 15.4 Mio t CO2 over 20 years. This corresponds to a yearly potential of 770 kt CO2 per year.
These estimates come with a great caveat: carbon stocks tend to reach a saturation point. Once the saturation point is reached, further carbon inputs cease to translate into greater soil carbon content. Beuttler et al. (2019) estimate that at the rates here considered, soils would saturate after about two decades. However, the estimate of the saturation time is uncertain. For example, West and Post estimated that across 67 long term experiments the time to saturation for soils under crop rotation and no-till practices is around 15 year (FOEN 2019d). Smith estimates that soil carbon saturation ensues after 10-100 years, depending on soil, climate and SCS characteristics (FOEN 2019d). The IPCC uses a default saturation time of 20 years (Griscom et al. 2017). The total cumulative sum for SCS in soils would then amount to 37.4 Mio t CO2 in twenty years (Lewis et al. 2019). This corresponds to about a full year of emissions in Switzerland. For deep ploughing, the total cumulative SCS over two decades would amount to 15.4 Mio t CO2 (Lewis et al. 2019). Furthermore, SCS practices would need to be maintained in order to avoid losing carbon back to the atmosphere: thus, a risk of reversion of the carbon gains exists if practices are not stabilized. This also means that costs associated with SCS practices will persist once soils have saturated.
Global SCS potentials are orders of magnitude larger than Swiss potentials. Fuss et al.’s literature review over twenty three different studies gives an estimate of a mean global SCS potential of 4.28 Gt CO2 per year and a median potential of 3.68 Gt CO2 per year (Griscom et al. 2017). This corresponds to about 9-11% of current global emissions. A more recent estimate by Lal gives a much higher potential of about 9 Gt CO2 per year, corresponding to about 23% of global emissions per year. Lenton estimates that a maximum yearly potential of about 3.3 Gt CO2 per year may be achieved for ca. 12.5 years (Hofer et al. 2007). It should be noted though, that due to saturation effects and possible re-release of carbon after cessation of SCS practices the total cumulative potential of SCS is limited.
Financing
Several different estimates do exist, but these depend strongly on geographic location and soil composition. In a review, Fuss et al. (2018) report only three papers that provide estimates for the cost of SCS, namely (FOEN 2019d) (Schwaab et al. 2015). According to Smith’s estimates, about 20% of global SCS could be realized at negative cost, ranging from -45$ to 0$ per t CO2 eq. About 80% could be realized at a cost between 0$ - 10$ per t CO2 eq (Griscom et al. 2017). Total costs for global implementation under these conditions would amount -7.7 B$. These estimates suggest great potential for scalability.
In Switzerland, the only cost estimate for SCS is given by Beuttler et al., and amounts to 0-80 CHF per t CO2 (2019).
To our knowledge, these estimates ignore the opportunity costs of carbon: The costs from climate damages incurred by not implementing SCS. These costs are substantial: Nordhaus estimates them to lie around 30$ per t CO2 (Hilaire et al. 2019).
Social Compatibility
The risks of SCS are manifold. The amount of carbon soils can sequester is limited. Influx rates saturate with the cumulative amount of sequestered carbon. Sequestered soil carbon can be re-released into the atmosphere if SCS practices are not maintained. Thus, benefits from SCS practices will provide ever smaller marginal benefits as soil carbon stocks reach saturation levels. Finally, there may exist trade-offs between minimizing CO2 emissions by SCS and minimizing other greenhouse gas emissions from soils.
However, as laid out in Fuss et al., there are substantial benefits to SCS. Most importantly, SCS does not compete for land with food production. SCS leads to improved soil quality and health (BAFU 2013) and improved and more stable crop yield (BAFU 2017). SCS’s water footprint over large land areas is estimated to be negligible (FOEN 2019d). Additional benefits are the reduction of environmental impacts of fertilization (smaller nitrate leaching and reduction of NO2 emissions), improved water retention and infiltration of the soil (by use of cover crops), reduced risk of erosion (cover crops) and improved drought resistance due to the use of deep rooting crops (Lewis et al. 2019). Several of these benefits are effective measures to combat climate change associated risks. Lastly, a central benefit lies in the scalability of SCS approaches: Well-tested approaches could be deployed globally. In particular, SCS appears to be the only negative emissions technology where a substantial fraction of possible adopters could implement SCS at an economic benefit. This adds to the scalability potential of the technology.
Questions and Uncertainties
- It remains uncertain how validation that soil has been sequestered in a particular agricultural field of interest (monitoring of successful sequestration) may be implemented. This is an active field of research, with progress being made (BAFU 2019).
- How can it be avoided that other greenhouse gases are emitted (for example N2O) instead of CO2? Where are there trade-offs? Can these trade-offs be overcome? Smith notes that many of the adverse effects constituting these trade-offs can be overcome with the appropriate portfolio of SCS techniques (FOEN 2019d).
- It is uncertain where the saturation levels for carbon retention lie given a specific type of soil.
- Reversibility of soil carbon sequestration efforts: Soil carbon sequestration is vulnerable to reversal if the land management techniques are changed in a detrimental way. It remains uncertain how fast the re-release would be. It remains uncertain how the lack of permanence may be addressed by different methods, for example by approaches increasing suberin in plant roots.
- The build-up of soil carbon requires added plant nutrient matter, in particular nitrogen, phosphorus and potassium (Griscom et al. 2017). Adding these without appropriate management techniques could lead to an exacerbation of fertilizer-associated leakage into water courses.
Synthesis
Comparison of Technologies
Potential and cost of negative emission technologies in Switzerland:

Conclusion
In order to limit global warming to 1.5 °C, the large-scale application of negative emission technologies is required (Hilaire et al. 2019), and this will be necessary as early as 2025 (IPCC 2018). In general, a stable global temperature can only be achieved if greenhouse gas emissions are net zero. For residual emissions that cannot be avoided (cement, aviation, chemical industry, agriculture) negative emissions are needed to achieve net zero emissions. The globally permissible CO2 budget for 1.5°C must not be exceeded: On one hand, this will likely cause irreversible damage to ecosystems and human suffering, and on the other hand, it would only postpone the problem, which is still solvable today, into the near future, thus leaving society with a huge mortgage, the future financing of which will cause major problems, as the polluters today can no longer be prosecuted. If Switzerland can take a leading position in the development of NETs, it would be able to provide other countries with the necessary NETs in the event of their CO2 budget being exceeded, thus making a major contribution to the global solution.
This chapter summarizes the most important techniques for extracting and storing CO2 from the atmosphere (NETs). The potential of NETs possible in Switzerland exceeds Switzerland's domestic and grey greenhouse gas emissions for the year 2018. The most promising technologies are Biochar, Soil Carbon Sequestration, Carbon Dioxide Capture (CCS) at point sources of CO2 such as cement plants and waste incinerators, and Direct Air Carbon dioxide Capture and Storage. All proposed technologies are considered to be very safe and have minimal environmental impact.
The necessary technologies are already relatively advanced and several of them could be operated in Switzerland:
Direct Air Capture and Storage
With Direct Air Capture, CO2 is extracted from the ambient air using technical equipment. The CO2 thus extracted in Switzerland is safely stored (sequestered) in the earth's crust, either in Switzerland (capacity expected to be 2.68 Gt), or abroad (capacity according to IPCC certainly over 2000 Gt - with global emissions of approx. 40 Gt annually). The storage of CO2 in soil has been going on for 40 years, so far about 260 Mt CO2 (Global CCS Institute 2019). It is considered very safe. Alternatively, Direct Air Capture could also be operated abroad to avoid the transport route and benefit from cheap renewable energies.
Carbon Storage and Carbon Usage
By burning biomass (e.g. plant waste, wood residues, etc.), heat or electricity can be generated and the CO2 emitted from the exhaust gases can be stored in the ground, as with DACCS. This allows carbon to be removed from the carbon cycle and safely stored.
Carbon Capturing
In industrial point sources such as waste incineration plants or cement production, CO2 can be filtered out in a targeted manner due to its high concentration and stored in the ground as with DACCS.
Enhanced Weathering
In the process of Enhanced Weathering, crushed mineral rocks are distributed over fields. By crushing the rock, it reacts more quickly with the CO2 bound in the rainwater - its natural weathering process is thus accelerated. Washed into the sea via water bodies, the CO2 is stored there as carbonate rock for the long term. This process thus also counteracts ocean acidification. The potential in Switzerland lies around 2.5-4.0 Mt CO2/year with a price of 160-190 CHF/t CO2.
Afforestation, Reforestation and Wood Usage
Through reforestation, forest management and increased use of wood in buildings, up to 3 Mt CO2 can theoretically be stored in Switzerland each year. Afforestation has very limited potential in Switzerland.
Biochar
It is also possible to convert fast-growing plants or waste from food production into vegetable carbon under great heat and then store it in the soil. The waste heat can be used directly or converted into electricity.
Soil Carbon Content/ Sequestration
Changes in agricultural land use can also increase the carbon content of soils, which would also improve soil quality.
Many of these technologies could store between two and three megatons of CO2 per year in Switzerland. Depending on the technology, the price is between 50 and 300 CHF per ton of CO2 and, in the case of soil carbon sequestration, could even lead to an increase in agricultural yields that exceeds the price of the resources used. At the moment, however, the prices of these technologies are much higher due to a lack of demand. In order to achieve sufficient development and application, NETs must be financially supported. Various political strategies for implementing these technologies are listed at the beginning of the main part of this chapter (2. Negative Emission Methods).
"Call to action"
Negative emission technologies (NETs) are practically indispensable to meet the 1.5 target for two reasons:
- They allow processes for which no CO2-free alternatives currently exist to continue to be used. These include cement production, air traffic, agriculture, the chemical industry, etc. Without NETs, these areas would have to be completely shut down or converted to synfuels by 2030, but their production is many times more costly compared to NETs.
- Switzerland must be prepared for the fact that net negative emissions (more remote than emitted CO2) will be needed if the global CO2 budget is exceeded - we must therefore invest in these technologies as soon as possible.
NETs need the support of politics and society - they will not be able to assert themselves on their own; this would require either draconian laws or very high CO2 prices. It makes much more sense to ramp up NETs with government aid, while the learning curve makes these technologies cheaper and cheaper and within about a decade a CO2 tax is reached which is then twice the cost of NETs - net zero would be reached.
NETs enable net zero to be implemented in a socially and economically acceptable way - but they are in no way a free ticket for "business as usual" and should therefore be combined with measures that are part of the CAP and described in the other chapters.