Energy Supply
In this chapter we show how the transition to a fossil-free energy supply could look like.
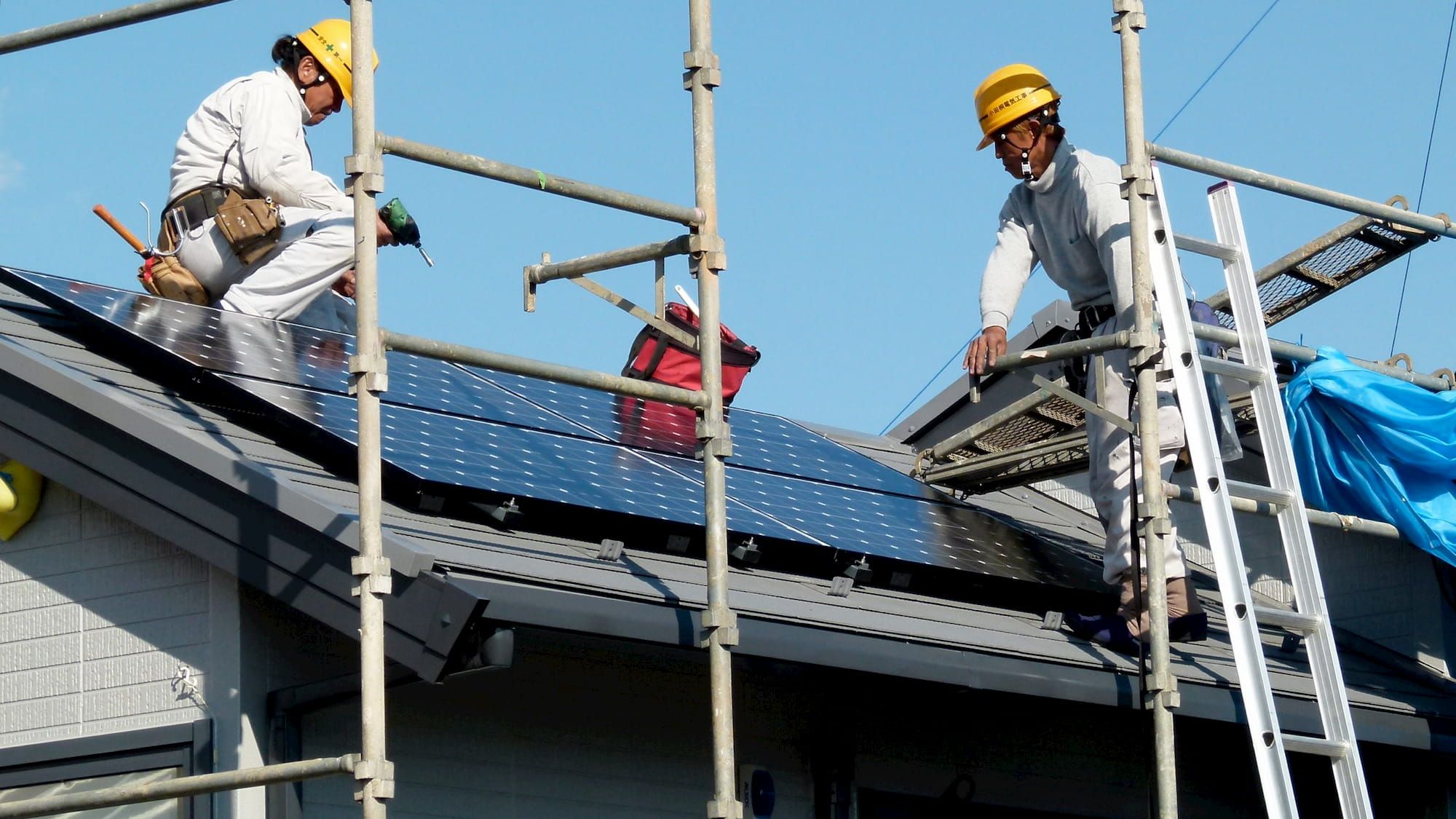
You are reading the full version of the Climate Action Plan. Do you just want to read the Executive Summary? Click here for the Executive Summary:
Some abbreviations are used in this chapter. You can find explanations of all abbreviations in the glossary.
Vision
Introduction and Aim
Three quarters of Switzerland’s greenhouse gas emissions are energy-related, mainly originating from the burning of fossil fuel-sources (SFOE 2020). Therefore, the transition to a fossil-free energy supply, the so-called decarbonization of the energy system, is of enormous importance. It is expected that this will lead to an increase in electricity demand, like through the transition to electric mobility and through the deployment of heat pumps for the heat supply of buildings. Power represents the main pillar of a fast and profound decarbonization of the Swiss energy system as electrification directly leads to decarbonization in many sectors. Other sources of renewable energy, i.e. renewable heat and renewable fuels, are covered in other chapters of the CAP.
The following sections will, firstly, estimate future electricity demand until 2030 and 2050 and describe the current political situation. Afterwards, different political instruments will be highlighted, which should precipitate the necessary development of renewable energies together with grid and storage technology. Finally, several technologies and their development potential will be covered in greater detail.
Future Electricity Demand
The succeeding figures refer to the target year of 2030 or 2050 for the achievement of a complete decarbonization in the sectors of mobility (without international air traffic) and building energy. The transition from today until 2030 or 2050 in the energy source’s composition has been linearly presumed, respectively. The large part of the increased electricity demand in 2050 as opposed to 2030 comes from the commitment to electricity from nuclear energy. It has been assumed that the remaining nuclear power plants between 2030 and 2040 will be gradually decommissioned.
It will be differentiated by two different scenarios:
a) The ‘Growth in Accordance with the State’ Scenario
The official prognoses of the State will be applied for population growth, movement of goods, people per kilometer, heat demand and so forth. The most important assumptions are:
- Increases in the mileage in mobility according to Wüthrich et al. (2017), with no sufficiency measures.
- The renovation rate in the building sector continues at 1% annually.
- The exhaustion of the whole development potential in accordance with the OFE for hydro energy (which results in an additional 2.2 TWh annually) and biomass (which results in an additional 3.2 TWh annually) for electricity production until 2030, instead of until 2050.
- The exhaustion of half of the efficiency potential in the current range until 2030 (instead of 100% until 2050). This results in power savings of 9 TWh annually until 2030.
- The exhaustion of a third of the wind power potential in accordance with the OFE until 2030 and of the full potential until 2050. This corresponds to a production of 1.5 TWh annually with approximately 300 new wind turbines until 2030 and 4.3 TWh annually with approximately 800 wind turbines, respectively.
For details of the assumptions see (Sperr and Rohrer 2019).
b) The ‘Sufficiency and Efficiency’ Scenario
The implementation of sensible measures in other sectors (see chapters on mobility and buildings) can clearly reduce electricity consumption. In comparison to the assumptions made by the State (the "Growth in accordance with the State" scenario), the following modified assumptions were made for the development of energy demand:
- The mileage in mobility declines by 20% until 2030 compared to today in all aspects and, therefore, stay constant until 2050.
- The renovation rate in the building sector amounts to a constant 3% annually from 2020
All other assumptions like, for example, population development or living space have been adopted by the State as it stands and are, consequently, identical with the scenario; ‘Growth in accordance with the State’.
An Estimation of the Additional Electricity Demand
Subsequently, the decarbonization of mobility and the heat production for buildings is being contemplated. The chosen technology-mix is leading to an increase in electricity demand. Table 5‑1 shows how strong the production from photovoltaics facilities must be developed with both scenarios, in order to receive a balanced annual budget from electricity. The numbers originate from the, still, unpublished decarbonization calculator of the Zurich University of Applied Sciences, Wädenswil, as well as from (J. Rohrer and Sperr 2018; Sperr and Rohrer 2019; 2018).
Table 5-1: additional electricity demand from the decarbonization of mobility (without international flight traffic) and of the heat production for buildings like; potentialities for energy savings and the development of the electricity supply without photovoltaics. The difference between demand and potential must be covered either through photovoltaics or through imports. An annual operating figure of 3 has been assumed with the heat pumps (HP).
Scenario | Growth in accordance with the State | Sufficiency and Efficiency | |||
Year: | 2030 [TWh] | 2050 [TWh] | 2030 [TWh] | 2050 [TWh] | |
Cars 100% electric | 14.2 | 15.4 | 10.9 | 10.9 | |
Delivery vehicles 100% electric. | 2.74 | 3.46 | 2 | 2 | |
Truck/LKW 80% elektr., 20% hydrogen | 4.74 | 5.7 | 3.5 | 3.5 | |
Buildings 80% HP, 20% wood / solar | 18.6 | 15.8 | 15.9 | 11.4 | |
Replacement of nuclear power | 22 | 22 | |||
Total demand | 40.28 | 62.36 | 32.3 | 49.8 | |
Power efficiency, half of potential | 9 | 9 | 9 | 9 | |
Hydropower | 2.2 | 2.2 | 2.2 | 2.2 | |
Biomass | 3.2 | 3.2 | 3.2 | 3.2 | |
Wind energy | 1.5 | 4.3 | 1.5 | 4.3 | |
Remaining for PV | 24.38 | 43.66 | 16.4 | 31.1 |
The above known figures concern the annual operating figures. Decarbonization within industry, agriculture and air traffic have not been considered. The transformation and storage-losses would still have to be counted amongst that, concerning a storage of electricity (day/night or seasonal). This concerns battery storage of 10-15 %, pumped-storage power plant of 25-30% and storage in the form of gas at 60-70%.
Current Situation
Existing Policies
Switzerland was a pioneer for the development of renewable energy and hydropower was developed early on. Today, Switzerland belongs to the ‘late bloomers’, with reference to the development of new renewable energies internationally. The strengthened use of renewable energies was constituted in the Energy Act of 1998, but the policy of encouragement was always very restrained. Therefore, Switzerland adopted the success model of the German Renewable Energies Act not until around 10 years later and then implemented it in such a way that the development was strongly limited through financial limitations (the means originate from a limited network supplement). Consequently, mainly waiting lists were established. The phasing out of nuclear power was put into law with the energy strategy of 2050, which was drawn up by the State after the Fukushima nuclear disaster in 2011. However, the replacement with renewable energies has, again, only been addressed hesitantly. The highly limited effectiveness of feed-in tariffs has been limited in time and replaced by one-off investment contributions (again with financial limitation). In the longer term, the 2050 energy strategy relies on imports: the statutory objectives, which are hardly being achieved with the existing measures, can replace no more than half of the omitted electricity generated by nuclear power. Additional demand through electrification over the course of decarbonization is not even considered.
A further development of the Energy Strategy is currently in planning. The Federal Council has started a consultation legislative process for the Energy Law in April 2020, in order to slightly adjust the financial measures. However, the financial constraints are remaining in force, currently at 2.3 centimes grid surcharge per kilowatt hour, which not only finances renewable energies, but also efficiency and water protection measures. One-off investment contributions will continue to be the focus for all technologies. In most EU countries, however, there is a payment for the fed-in kilowatt hour (floating market premiums) for a specific period of time, for example 20 years. This offers a clearly higher investment security than with one-off payments.
Likewise, the revision of the Electricity Supply Act is pending. The tariffing of the network will be an issue there. However, the government, still, does not want to incorporate the topic of distance-dependent network tariffs, where only the network levels that are actually used are to be paid for. Today, a consumer who draws electricity from the neighborhood pays grid charges for all 7 grid levels instead of only for the lowest grid level.
Policy Measures
In the following, we propose a set of eight policies which we expect to be most suitable to achieve the outlined renewable energy and storage targets. As we do not expect all proposed policies to be promptly adopted, some policies overlap in their scope. However, we recommend the implementation of as many policies as possible to maximize the greenhouse gas emissions reductions that other sectors may achieve thanks to electrification.
Technologies and Potentials
After estimating future electricity demand and discussing the political action necessary to ensure energy supply in a net zero world, we want to take a closer look at the potentials and technologies necessary for this transition.
Photovoltaics
This section as well as parts of the section on future electricity demand were already developed further by the author and published via the Swiss Energy Foundation (see Jürg Rohrer 2020).
Photovoltaic systems have, by far, the biggest potential for increased electricity production in Switzerland (Sperr and Rohrer 2018), particularly during the short time span of 10 years. In this section, therefore, it will be estimated, how rapidly and strongly the electricity production from photovoltaics in Switzerland could be developed and how, through this, a complete supply of electricity would be possible in the annual budget.
Potential of PV Roof Surface Area in Switzerland
Studies by (Gutschner, Gnos, and Nowak 2010; Nowak, Gutschner, and Gnos 2007; Nowak and Gutschner 2011) have shown that roughly 30% of the roof surface area in Switzerland would be suitable for the installation of a solar PV installation. In a first approximation the roof surface areas are being estimated by the means of the floor area of 485km2 (Walch et al. 2020). This then corresponds to a modular unit surface of 145.5km2; and assuming the values of 0.188 kWp/m2 and 970 kWh/kWp for said unit one gains 26.5 TWh per year. This potential has been put to use in an online potential calculator for renewable energies per municipality (Eymann, Rohrer, and Stucki 2014).
According to the data of sonnendach.ch roof surface area in Switzerland rated good, very good or outstanding has a PV potential of 77.8 TWh per year. After subtracting 5% to account for protected buildings (Remund, Albrecht, and Stickelberger 2019) one is left with 74 TWh per year. According to a clarification by (Portmann et al. 2019) one ought to subtract 30%. When it comes to flat-roofed blocks of flats, the subtrahend amounts to 58%. These deductions account for chimneys, skylights, terraces, railings, roof structures etc. Since 6% of the gain comes from blocks of flats the average deduction is 32% (94% with 30% and 6% with 58%). These deductions have been defined through expert surveys. However, the exact methods and the raw data have not been published (Portmann et al. 2019). Other studies concluded bigger deductions i.e. around 40-45% (Assouline, Mohajeri, and Scartezzini 2017; Assoulinea, Mohajerib, and Scartezzini 2018; Walch et al. 2020).
Thus, the data from sonnendach.ch results in a roof surface area potential anywhere between 40-50 TWh per year. Due to the aforementioned data, the Swiss Federal Office of Energy (BFE/OFEN/UFE) assumes a potential of 50TWh (BFE, 2018). The solar branch association Swissolar calls this the exhaustible potential and finalizes a similar value of 49.1 TWh. However, Swissolar assumes that only half of this value can be harnessed in the next thirty years i.e. 24 TWh per year (Remund, Albrecht, and Stickelberger 2019).
A new study conducted by the EPFL (École polytechnique fédérale de Lausanne) put the number of said potential to solely 24.6 (± 9) TWh per year (Walch et al. 2020). Main reasons for said difference, especially concerning the estimates of sonnendach.ch, are thought to be different models of insolation, the machine-aided arrangement of the modular units on the roofs and more detailed calculations of the shading (Walch, Mohajeri, and Scartezzini 2019). Notwithstanding, the immense differences are still not sufficiently explained.
At first sight, the estimates for Swiss roofs seem the cover a wide range i.e. 24.6-49.1 TWh per year. What meets the eye is the high value stated by sonnendach.ch when compared to the others (see Figure 5‑1). However, assuming that only half of the potential could be harnessed until 2050 (in compliance with Remund et al., 2019), one gets a similar potential to the other estimates. Nonetheless, further clarifications are suggested.
Since the time horizon of this report refers to 2030 or at most 2050, an exhaustible potential somewhere between 24-25 TWh on roofs seems to be the most trustworthy and sensible estimate. To this figure one can add Swissolar’s estimate of 8 TWh for façades. Hence Swiss buildings might be producing 30-33 TWh worth of energy per year.

Analysis of Solar PV on Roofs
The size of a solar plant is a decisive factor when it comes to the costs of said structure and, thus, also influences the production costs of electricity. In-detail data has only been published by sonnendach.ch. Hence, the following analysis is based on their information. As long as the factor 0.5 is being used for the number and the surface area resp., the statements should be transferable to the aforementioned trustworthy estimate of the potential i.e. 24-25 TWh.
For the analysis it has been assumed that several roof surface areas of one building would be conflated to one single solar plant. Thus, different surface areas with an identical UUID have been added up and labeled as “solar plant” or “building”.
Furthermore, only roof surface areas with an annual insolation of at least 1,000 kWh/m2 have been taken into account. This is identical with a very good or outstanding roof surface area.


Figure 5‑2 shows the standardized annual gain of the solar collectors on roofs as per sonnendach.ch. Most of the collectors are in the range of 900-1,000 kWh/kWp, as are newly installed collectors today.
Figure 5‑3 shows the distribution of the sizes of the roof surface areas from sonnendach.ch, which are usually fit to have PV systems installed. Figure 5‑4 shows the annual electricity yield sorted by the size of the installations. The abundance distribution of the annual electricity yield for different categories of PV systems are shown in Table 5‑2 and in Figure 5‑5, also. As a data source for the categorization the figures from sonnendach.ch, with a lump reduction of 30% of the roof surface area and the gains respectively, have been used. Furthermore, the roof surface areas were also aggregated with the UUID to PV systems.

Table 5‑2 as well as Figure 5‑5 show nicely that typical installations on single-family homes with capacities between 2-20 kWp (10-100 m2 surface of modular units) can produce roughly 36% of rooftop PV electricity. 29% of the potential comes from installations between 20 and 50 kWp and 25% of the potential is being covered by even bigger installations on blocks of flats or on industrial builds.
These bigger installations can be built more efficiently than small ones and, thus, lead to substantially lower electricity production costs. Especially when a lot of solar power must be added in a short time, the political measures should be adjusted to this circumstance. This calls for political measures that will quickly lead to the construction of PV systems on larger roofs.
Table 5-2: Frequency of the annual PV gains (source of data being sonnendach.ch grouped by UUID with a correcting factor of 0.7 as a lump deduction e.g. for chimneys, roof structures etc.)
Category [MWh] | Electricity yield [GWh] | Area [km2] | Number of plants |
<2 | 329.31 | 2.01 | 266'052 |
2 - 4 | 1'232.27 | 7.45 | 424'647 |
4 - 20 | 18'945.87 | 111.15 | 1'835'675 |
20-50 | 15'156.99 | 90.48 | 520'871 |
50-300 | 13'302.59 | 80.61 | 149'218 |
>300 | 5'578.92 | 33.86 | 8'908 |
Total | 54'545.96 | 325.57 | 3'205'371 |

Potentials Outside of Buildings
Studies for the assumptions of potentials of the PV-production independent from buildings in switzerland have rarely been published so far. Swissolar and Meteotest assume the following additional potentials, which could be realised in the next 30 years (Remund, Albrecht, and Stickelberger 2019):
Plant | Potential in TWh | Area km2 |
Streets | 2.5 | 16.2 |
Parking lots | 3.9 | 25.7 |
Motorway embankments | 3.9 | 25.7 |
Total: | 10.3 | 67.6 |
Additionally to this potential on existing infrastructures from 10.3 TWh per year, there is a potential of plants in the lowlands and that mountains that is theoretically only limited by the Area of Switzerland. However, the authors of the study mentioned above assume only a limited potential of 3.3 TWh due to assumptions about the distance of feeding options in the Alps (Remund, Albrecht, and Stickelberger 2019).
Comparison of Potential and Requirement
According to Table 5‑1, there is a requirement of additionally 24.4 TWh PV-Energy per year until 2030 respectively 43.7 TWh PV-Energy per year until 2050 in the scenario «Growth in Accordance with the State». The required PV-Energy was determined with optimistic assumptions concerning the realization of Power-efficiency measures and the expansion of the other technologies for the production of energy (Wind power, Hydropower, Biomass). These requirements in energy are put next to the potentials for PV-energy shown in Table 5‑3. In that case, it is assumed that financial and human resources represent no bottlenecks (see also considerations down below) and that the potentials can be fully implemented until 2030 respectively 2050.
To implement the PV-potentials that were identified completely and in a short time span, rigorous political action is necessary. Figure 6 shows the comparison between the demand for PV electricity of around 44 TWh in 2050 and the forecast PV production, assuming different degrees of exploitation of the potential. Even a full exploitation of the PV potential by 2050 on existing infrastructures could not completely cover the needs in the "growth according to the federal government" scenario. In the “sufficiency” scenario, on the other hand, a 70% exploitation of the potential on infrastructures or a full exploitation of the PV potential on buildings (roof areas and facades) would be sufficient to cover the demand.
So far, only the annual balance of electricity demand and production have been taken into account. Due to the seasonal course of PV production in the Swiss plateau and the high proportion of PV electricity, seasonal electricity storage will most likely be necessary. Regardless of the storage technology chosen, storage and reconversion are associated with losses, so that ultimately the electricity consumption will be higher. In addition, neither any additional electricity requirements due to decarbonization in industry nor for the substitution of aviation fuel have been taken into account.
This makes it clear that consistent sufficiency measures to reduce electricity requirements and / or PV systems on open spaces will be necessary to cover Switzerland's electricity needs in 2050 in the annual balance sheet.
Seasonal storage: A forced expansion of PV systems in the mountains and wind power would reduce the seasonal storage requirements. Otherwise, depending on the efficiency of the selected storage technology, much larger free areas will have to be covered with PV systems.
Table 5-3: Comparison between the demand per scenario and the potential for PV electricity on existing infrastructure.
in 2030 | 2050 | ||
Production potential of PV electricity: | |||
PV on roof areas | 24.5 | 24.5 | TWh |
PV on facades | 8 | 8 | TWh |
PV on parking lots, roads, embankments | 10.3 | 10.3 | TWh |
Subtotal PV-Production Potential | 42.8 | 42.8 | TWh |
Scenario «Growth in accordance with the State» | |||
Electricity demand from PV | 24.4 | 43.7 | TWh |
Requirement minus potential | 18.4 | - 0.9 | TWh |
Scenario «Efficiency and Sufficiency» | |||
Electricity demand from PV | 16.4 | 31.1 | TWh |
Requirement minus potential | 26.4 | 11.7 | TWh |

Personnel Requirements and Training of Additional Personnel
The annual rate of expansion of photovoltaics in Switzerland is currently around 350 MW (Hostettler 2019). Additional specialists - especially PV planners - need to be trained to add more quickly. Recruitment and training take time, which is considered the most important limiting factor.
According to the “Strategy for Solar Education in Switzerland”, a distinction is made in the solar industry between expert knowledge (3% of employees), specialist knowledge (25% of employees) and basic knowledge (72% of employees) (Portmann et al. 2017). In the case of tenders for PV systems, a distinction is normally made between the hours worked for planning or building the system. Around 17% of the working hours are devoted to planning, the remaining 83% for the construction of the plant (Sperr and Rohrer 2017; 2018). In the following it is therefore assumed that a 1-2 week “apprenticeship” is sufficient for 85% of the work and that only 15% of the work is carried out by well-trained specialist planners. These specialist planners have a solid basic technical education (technical school level or university of applied sciences level), special training lasting around 2 months and additionally at least half a year of work experience in the planning of PV systems.
Due to the necessary training of additional specialist planners, the PV expansion until in 2030 will be limited to approx. 27 TWh (see Figure 5‑7). The average investment costs of a PV system in 2020 were CHF 1,200 per kWp, a future cost reduction of the PV systems of 1% per year and a share of the installation and planning costs in the investment costs of a PV system of 30 % are being assumed (Sperr and Rohrer 2017). This enables the costs for the work performed during the construction and planning of the PV systems to be calculated. The gross income per full-time equivalent for planning and installation in the PV industry is CHF 67,000 per year (Huemer 2016). Ancillary wage costs, tools, vehicles, office rent, administration, etc. are taken into account with a factor of 1.5, so that costs of CHF 100,000 are charged per FTE. As mentioned above, the costs for specialist planners make up 15% of the total labor costs for planning and building a PV system. The number of required PV specialist planners in Figure 5‑8 was thus determined.
This leads to an increase in the need for specialist PV planners from around 200 full-time equivalents today to around 2,500 full-time equivalents by 2030 (see Figure 5‑8). For the peak year 2031, there will therefore be a need of:
- Ca. 2'500 specialist planners
- Ca. 17'000 Workers in mounting
The training of 2,300 specialist planners within 10 years should not be an insurmountable problem. The only question is whether so many people can be motivated for this job. The assembly staff could be temporarily supplemented, for example, by the army or civil service.
For comparison: there are currently around 5 million employees in Switzerland. In sector G, “Trade, maintenance and repairs of motor vehicles”, the transition to public transport and electromobility is likely to result in some redundancies. Currently, 603,000 people are employed there in Switzerland. The approximately 20,000 persons mentioned above represent 3.3% of the employees in Sector G.


Wind Energy
Current Situation of Wind Energy in Switzerland
At the end of 2019 there were 37 wind turbines installed in Switzerland with a total installed capacity of 75 MW. This includes the wind farm Juvent in Western Switzerland with a total of 16 Vestas wind turbines (four V112 and 12 V90 models). The total electricity production from wind energy was 146 GWh – equal to less than 0.3% of the total production in Switzerland. The development of the installed capacity (red), electricity production (dark blue) and expected production (light blue) since 2005 is shown in Figure 9. A further 11.75 MW are expected in 2020, when the wind farm San Gottardo starts production, making a total of 86.75 MW and 42 wind turbines.

Wind Energy Potential in Switzerland
According to the Energy Strategy 2050, Switzerland aims to produce 4.3 TWh of electricity from wind energy by 2050, corresponding to approximately 800 new wind turbines with a total new installed capacity of 2.8 GW assuming an average installed capacity of 3.5 MW and 1'500 full load hours (Cattin et al. 2012). However, more recent studies suggest that the potential would be on the order to 9 TWh. On the other hand, problems with permitting due to acceptance issues have slowed down this process, and not a single wind turbine was installed in 2018.
Wind Energy Production by 2030
Assuming a linear increase of installed capacity between 2020 and 2050 to reach the Energy Strategy goal by 2050 results in an estimated installed capacity of 267 wind turbines with 933 MW by 2030, and an electricity production of about 1.5 TWh. Through rapid implementation of ambitious measures (e.g. cantonal electricity quotas, simplified permitting process) this number could certainly still be raised.
Efficiency
The main drivers of electricity consumption are electrification for building heating and mobility. Efficiency and sufficiency measures in these areas are covered in the corresponding chapters (see Chapter 2: Mobility and Chapter 4: Buildings).
Additional reduction of energy consumption is possible in other areas. In addition to sufficiency, the savings potential purely through technical progress, such as optimized heating circulation pumps, energy-efficient refrigerators and freezers or LED lighting, is around 26 TWh (Schweizerische Agentur für Energieeffizienz 2011).
Table 5-4: Electricity efficiency potential for Switzerland. Scenario TECHNIK describes the demand with growth factors (population growth, jobs, replacement of fossil fuels, etc.) and the exploitation of the technical potentials. (Source: Schweizerische Agentur für Energieeffizienz S.A.F.E., 2011)

Whenever devices are replaced before the end of their service life, the grey energy must be considered - so it may make sense to operate a less efficient appliance for longer, depending on the purpose. This also applies to a certain extent to fossil appliances (cars, heaters), although here replacement with electrical alternatives makes sense as soon as electricity from renewable sources is available.
Power Grid
Source of texts and figures for this section: BFE 2015, Entwicklung der Netzkosten in der Schweiz vor dem Hintergrund des derzeitigen Bedarfs, der ES2050 und der Strategie Stromnetze.
Power grids must be continuously maintained and renewed. These measures alone generate costs, the amount of which is relatively easy to estimate, as they can be derived with a good approximation from today's - well known - installed assets. In addition, the Message on the first package of measures of the Energy Strategy 2050 from autumn 2013 and the Explanatory report on the electricity grids strategy from 2014 describe the changes that are being targeted in the areas of electricity generation, electricity consumption and power grids. These changes will in some cases have a significant impact on the future design and thus the costs of power grids - beyond the costs of maintaining existing grids.
Based on the study (Prognos 2012), this section describes the assumptions of the Energy Strategy 2050 (ES 2050) and the resulting estimates of future grid investment costs. These values can be taken as a rough estimation for the grid costs until 2030 if the net 0 CO2 goal has to be reached until 2030.
Assumptions of the Energy Strategy 2050
The current public and political debate on the future energy supply in Switzerland requires a comprehensive basis for decision-making. In the area of electricity supply, there is a need for a well-founded estimate of the costs of the power grids, which play a key role as the link between producer and consumer, in the coming decades.
In the past, various studies have been carried out to estimate future grid costs in Switzerland (Ladermann et al. 2010; Consentec 2012). However, some of these studies were based on assumptions that deviate from the current boundary conditions set out in the ES 2050 and the Electricity Grid Strategy. Furthermore, the previous studies did not yet take into account the extensive cabling of the distribution grids planned in the Electricity Grid Strategy (Consentec 2013). A possible expansion of grid level 3 was also left out in the past. Furthermore, the time horizons of consideration as well as the presentation and differentiation of the results in the various studies are not uniform. Given this background, the future grid costs will be quantified again.
The ES 2050 describes, among other things, the targets for electricity generation and consumption in Switzerland for the next 35 years and outlines the measures planned to achieve them. On the basis of these descriptions, Prognos was mandated by the SFOE to derive various forecasts for electricity generation (supply variants) and for electricity consumption (demand scenarios). In the actual report (Prognos 2012), the supply variants E / C + E / C+ D + E and the demand scenarios wwb / POM / nEP / are considered.
Demand scenario wwb:
The reference scenario “continue as before” (dt. “weiter wie bisher” / wwb) shows the situation if all energy policy instruments currently in effect are not changed. The scenario results in an increase in electricity demand from currently around 59 TWh/a to 69 TWh/a in 2050 and thus in a higher load on the grids in consumption-dominated areas.
Demand scenario POM:
The scenario " Political measures" (POM) shows how the measures of the first package of the ES 2050 will affect energy demand. In this scenario, electricity demand is expected to increase slightly to 61 TWh/a in 2050.
Demand scenario nEP:
In the scenario "new energy policy" (nEP) a development of energy consumption is presented, which makes it possible to reduce CO2 emissions until the year 2050. This scenario leads to a slight decrease in electricity demand to 53 TWh/a in 2050 and thus to a slight relief of the grids in consumption-dominated areas.
Supply variant E (renewable energies):
In this variant, no additional central large-scale power plants are built. Instead, an ambitious expansion path for renewable production facilities is assumed, based on a corresponding political support regime. In the referred study, the expansion of decentralized combined heat and power (CHP) plants is based on the current support regime. The installed renewable energy capacity is approximately 2 GW in wind systems and approximately 10 GW in photovoltaic systems in 2050. The installed capacity of decentralized CHP systems is approximately 4 GW. Depending on the demand scenario, generation from decentralized generation plants and large hydroelectric power plants in Switzerland will not be sufficient to fully meet Swiss electricity demand. In this supply variant, the remaining shortfall is filled by imports.
Supply variant C + E:
In this variant, the same ambitious expansion path for renewable energies is assumed as in variant E. The remaining coverage gap is not closed by imports, but by central gas and steam turbine plants in Switzerland. Here, depending on the demand scenario, 4 to 7 gas and steam turbines with an installed capacity of approx. 550 MW each are assumed. For decentralized CHP plants, as in variant E, current support mechanisms are assumed. Compared to variant E, the changes in the generation structure primarily affect grid level 1 (transmission grid). The feed-in-related load on the distribution grids is identical in variants E and C+E.
Supply variant C + D + E:
In this variant, the same ambitious renewable energy expansion path is assumed as in variant E and C+E. However, here the electricity gap is covered by a combination of additional decentralized CHP plants and central combined cycle power plants. To realize this, it is assumed that the support conditions for CHP plants will change accordingly. Depending on the demand scenario, between approx. 6 GW (scenario nEP) and approx. 7 GW (scenarios wwb and POM) will be generated in CHP plants in 2035 and between approx. 4 GW (scenario nEP) and approx. 8 GW (scenarios wwb and POM) in 2050. The number of CCGT plants is reduced here to 3 (scenarios POM and nEP) to 5 (scenario wwb). From the point of view of the distribution grids, this variant is expected to have the greatest load from feed-in. From the point of view of the transmission grid, a similar feed-in-related load is to be expected here as in the C + E variant.
Future Investment Costs
Below the expected need for investments in the Swiss grids for the period from today until 2035 and until 2050 is given. The demand includes investments in all grid levels, which result both from the forecast changes in supply and demand and from the replacement of the current grid infrastructure due to ageing. It is generally assumed that smart technologies in the form of adjustable local grid transformers will also be used.
Furthermore, the cost calculations for grid level 1 also take into account the multi-year planning of the national grid company Swissgrid (as of 2010). In the meantime - during the term of this study - Swissgrid has updated this multi-year planning and published a "Report on the Strategic Grid 2025". Due to time constraints, this new report could no longer be included in the calculations.
The results presented below also include investments for smart metering and the innovation budgets for intelligent grid solutions.
The presented results of the study (Consentec 2015) estimated grid costs until 2035 and 2050. These values can be taken as a rough estimation for the grid costs until 2030 if the net 0 CO2 goal has to be reached until 2030. More precisely, the grid costs for stock renewal until 2035 (which is needed anyway) and the grid costs for expansion until 2050 are used as benchmark in this report.
Investment costs with the Energy Strategy 2050
The need for expansion resulting from the measures according to the ES 2050 is illustrated below using the C+D+E supply variant. This supply variant can be regarded as a "worst case" variant. The results are differentiated according to the three demand scenarios wwb, POM and nEP. In the base case, it is assumed that controllable local grid transformers are used where they can reduce the need for grid expansion due to violations of the voltage range. Other smart expansion variants are not considered.
Table 5‑5 shows the calculated investment costs in the Swiss transmission and distribution grids until 2035 for the supply variant C+D+E and for the demand scenarios wwb, POM and nEP compared to the investment requirements without ES 2050. Independent of the supply variant and demand scenario, investment costs for the installation of smart metering components of approximately CHF 0.9 billion and innovation budgets of approximately CHF 235 million to CHF 255 million should be taken into account.
Table 5‑5: Investment costs [Billion CHF] until 2035. The smart meter costs do not include costs for ripple control systems (German: Rundsteuerung). If these costs were taken into account, the above costs would be about 10 % higher. (Consentec 2015)
The investment costs for the supply variant C+D+E are shown graphically in Figure 5‑10. In this supply variant, the additional costs compared to the case without ES 2050 are around CHF 14.4 billion in the demand scenario wwb, CHF 11.7 billion in the POM scenario and around CHF 9.3 billion in the nEP scenario. The higher investment costs compared to the C+E variant are mainly due to the higher expansion of decentralized thermal generation (CHP). Due to the higher expansion of distributed generation, the costs for cabling caused by the additional cost factor are consequently also higher. The additional costs caused by this are, however, rather low compared to the costs for cabling, which are needed anyway, and which are to a large extent determined by the replacement of the current grid infrastructure. In the wwb scenario, for example, they rise from CHF 4.7 to 5.2 billion. In contrast, the investment requirement for the cabling, which is caused by the load-related expansion and the renewal of existing infrastructure, is just as high as in the C+E scenario. If the grid is expanded purely conventionally (non smart), the investment requirement is CHF 1.5 billion higher in the wwb scenario, CHF 1.4 billion higher in the POM scenario and CHF 1.0 billion higher in the nEP scenario. Overall, therefore, by 2035 in the POM scenario and the C+D+E supply variant in the worst-case scenario, additional costs of CHF 13.1 billion will be required as part of the ES 2050 if only conventional expansion is implemented and smart metering systems and innovation budgets are included. However, an expansion that also makes use of controllable local grid substations is more likely (smart variant). In this case, CHF 11.7 billion can be expected by 2035.

Table 5‑6 shows the investment requirements in the Swiss transmission and distribution grids up to 2050 for the supply variant C+D+E and the demand scenarios wwb, POM and nEP compared to the investment requirements without ES 2050.
The cost base for smart metering components and innovation budgets, independent of supply and demand scenarios, will increase until 2050. For smart meters, this will be CHF 1.3 billion and CHF 410 to 445 million for innovation budgets.
Table 5‑6: Investment costs [Billion CHF] until 2050 . The smart meter costs do not include costs for ripple control systems (German: Rundsteuerung). If these costs were taken into account, the above costs would be about 10 % higher. (Consentec 2015)
The investment costs up to 2050 for the three demand scenarios in the C+D+E supply variant compared to the case without ES 2050 are shown in Figure 5‑11. In scenario wwb, the investment costs are CHF 21.5 billion higher than in the case without ES 2050. In the POM scenario these additional costs are CHF 18.2 billion. In the nEP scenario, the additional costs compared with the case without ES 2050 add up to CHF 10.7 billions and are thus only about CHF 0.8 billions higher than in the C+E variant.
What is interesting in the C+D+E variant is that the cost difference between the nEP and POM scenarios is much greater than in the C+E variant. Scenario POM in variant C+D+E is about 12 % more expensive than scenario nEP, while this difference is only about 7 % in variant C+E. This can be explained by the different expansion requirements resulting from the increase in decentralized generation plants. In the supply variant C+D+E, the expansion requirement increases by approx. 51% in the scenario wwb compared to 2035 and by approx. 59 % in the scenario POM. In the nEP scenario, however, the increase in costs is only approx. 39 %. In supply variant C+E, the expansion requirement in this period increases by approx. 33 % in scenario wwb, by approx. 41 % in scenario POM, and by approx. 52 % in scenario nEP due to the addition of distributed generation plants. This means that in supply variant C+D+E, the increase in expansion demand from 2035 to 2050 due to the addition of distributed generation plants is higher in scenarios wwb and POM than in supply variant C+E, whereas in scenario nEP this increase is lower in supply variant C+D+E than in supply variant C+E. This in turn can be explained by the different expansion paths for decentralized CHP plants in supply variant C+D+E. While until 2035 in all three demand scenarios CHP generation plants are built up to a similar extent, and this increase is continued in the demand scenarios wwb and POM until 2050, the decentralized CHP plants are reduced in the scenario nEP until 2050. This decommissioning in turn is caused by the decline in load and consumption predicted in the demand scenario nEP from today until 2050. The total expansion requirement for distributed generation plants is thus essentially determined by the expansion of distributed renewable generation plants. If the grid expansion were to be conventional (non smart), additional costs of approx. CHF 2.4 billion would be required by 2050 in the wwb scenario, CHF 2.2 billion in the POM scenario and CHF 1.5 billion in the nEP scenario.

Storage
Source of the texts and figures of this section: BFE 2013, Energiespeicher in der Schweiz, Bedarf, Wirtschaftlichkeit und Rahmenbedingungen im Kontext der Energiestrategie 2050.
In September 2013 the Federal Council presented planned measures within the framework of the Energy Strategy 2050 in a message to the parliament. Energy storage plays an important role within the framework of the Energy Strategy 2050. Since generation from stochastic energy sources does not necessarily correspond to consumption in terms of time, there is an increased need for interim storage of (electrical) energy. Energy storage must be further developed, on the one hand, by promoting research and, on the other, by adapting the regulatory framework for the electricity market.
To describe the potential contribution of storage technologies to the transformation of the power supply, the study (Hewicker et al. 2013) estimated the demand for different storage technologies in the context of the Energy Strategy 2050.
The study showed that, in addition to traditional pumped storage, several other technologies are now available or are expected to become commercially available in the foreseeable future. Most technologies are limited to short-term applications (hours to max. several days), with the exception of power-to-gas technology and potentially seasonal heat storage. The use of these technologies is limited in most cases by high capital costs, whereby a considerable cost degression is expected especially for battery storage.
In this section, a technology overview is given, and potential applications are shown. The required decentralized storage capacity in the distribution grid is estimated. Further demand for storage at system level is analyzed.
Technology Overview and Potential Applications
The storage of electricity is still dominated by pumped hydroelectric energy storage systems, which represent around 99 % of the installed capacity worldwide. This technology is well known and has been in use for decades, as is also the case in Switzerland. However, its expansion is linked to geographical conditions. In addition to pumped hydroelectric energy storage plants, compressed air storage systems, fly wheels and various battery technologies are now in commercial use worldwide. Other storage technologies are under development, some of them in an advanced phase, so that they will be commercially available in the near future. In the context of the study presented here, the following energy sources and technologies were considered: Chemical, kinetic, electrostatic, electromechanical, thermal.
In addition to the energy carriers used, the storage technologies differ in particular with regard to a number of technical properties. For the use in power supply the storage capacity (energy), the storage power, the efficiency as well as the reaction speed and the lifetime or aging are relevant. The storage capacity describes the maximum amount of energy that can be stored by the storage system, while the storage power describes the maximum charge/discharge power provided by the storage system.
In practice, a distinction is made between several power classes with regard to the size of a storage device, which refer to the maximum available discharge power. These range from micro-storage systems used in decentralized applications, through medium-sized storage systems at the medium and high voltage level, to large storage systems connected to the extra high voltage level. In the same way, various possible application areas for electricity storage systems can be identified. These range from ultra-short term storage in the range of a few seconds to minutes for voltage and frequency control, through classic daily storage for load leveling or balancing fluctuations in the feed-in of supply-dependent renewable energies, to seasonal storage.
As shown in Table 5‑7, unconventional power storage systems, with the exception of power-to-gas technology, are only suitable for short-term applications. The majority of potential storage technologies are therefore not available for medium to long-term storage, e.g. for balancing fluctuating production from supply-dependent renewable energies. In contrast, batteries in particular are very well suited for providing system services.
Table 5‑7: Overview of potential applications of storage technologies by power class and storage duration (source: “Energiespeicher in der Schweiz”, BFE 2013)

Decentralized Storage Systems
Especially for rural grids, a technically required storage requirement to avoid unacceptable grid overloads can be expected. This is particularly true in the case of a high level of additional decentralized, supply-dependent generation technologies, which will cause local overloading of the distribution grids with a comparatively high demand for decentralized storage solutions.
For distribution grids with a high load density, i.e. mainly in urban areas, on the other hand, no technical storage requirements could be identified. Due to generation close to consumption and a grid design for high loads, the urban grids considered in the modelling do not reach the limits of their capacity on the grid levels 6 and 7 or 4 and 5. Even the installation of electric vehicles does not lead to grid overloading given the assumed number of vehicles and charging strategies (an area-wide expansion of fast charging stations, which was considered in a sensitivity analysis, leads to overloads from about six fast charging stations per urban grid area and thus to theoretical storage requirements. However, in the case of grid overloads caused by electric vehicles, it is possible to use the storage integrated in the vehicle as mobile storage by means of intelligent controlled charging). Although in individual cases additional measures, e.g. to maintain voltage stability, may still be necessary, the expected storage requirement in distribution grids with a high load density can be estimated as low.
The highest storage demand was identified for the combinations NEP/E (and NEP/CE), as Table 5‑8 indicates. The combination of low load, caused by the expected effects of the efficiency measures in the demand variant NEP, combined with the high increase in renewable production capacity in the supply scenario E leads to a necessary storage power of up to about 1600 MW with a storage capacity (energy) of about 6600 MWh. The majority of the storage demand arises at the low voltage level. There, due to the grid design and the expected increase in decentralized production capacity, storage demand is mainly found in rural areas. If the power flow from the low voltage level to the medium voltage level is reduced at the low voltage level through the installation of storages, the result is only a small storage demand at the grid levels 4 and 5. This shows the different effects of the installation of storage systems compared to grid expansion. The grid expansion could enable higher power flows from the low voltage level to the upper voltage levels and thus the loading would be passed to upper grid levels. On the other hand, a storage system can reduce the loading close to the source.
Table 5‑8: Storage demand in the distribution grid according to scenario NEP/E (Energiespeicher in der Schweiz, BFE 2013)
Storage power [MW] | Storage energy [MWh] | |
Grid levels 6 and 7 Urban grids Suburban grids Grids in the mountains Rural grids | 0 200 310 925 | 0 560 1310 4330 |
Total | 1435 | 6200 |
Grid levels 4 and 5 Urban grids Rural grids | 0 180 | 0 400 |
Total | 180 | 400 |
Total needed storage in the distribution grids | 1620 | 6600 |
The values in Table 5‑8 are based on an estimate of a benchmark for the design of storage systems that are not designed to store all surplus energy, but can store about 2/3 of the surplus energy over the entire year. Furthermore, the stated storage capacities are to be understood as net values in the sense of a completely usable storage capacity. A reduction in the depth of discharge would therefore require a corresponding increase in overall capacity. With a depth of discharge of 80 %, an increase in the number of cycles of the storage systems could be achieved, and the storage capacity would increase by about 25 %.
Due to the number of cycles as well as the need for storage power and storage capacity (energy), battery storage is a suitable storage technology in the low voltage grid. In addition, the relatively small space requirement and scalability are advantages of a battery system. Battery storage systems have not yet been used in large numbers in low-voltage grids, but there has been an increase in the number of suppliers of storage systems for self-generated PV electricity as a result of developments in Germany.
Storage on System Level
In addition to the storage demand for the integration of the decentralized production technologies into the Swiss distribution grids, the further demand for storage was analyzed at system level. Under the assumption that the regulation of decentralized production due to an overfeed of the entire system should be avoided and all surpluses in Switzerland should be stored, a storage requirement at system level results. With the help of these storage facilities, surpluses can be absorbed, and it can be prevented that decentralized, renewable plants are curtailed due to an energy surplus. The excess energy is in particular due to the feed-in from wind and photovoltaic energy. In combination with the production of run-of-the-river hydroelectric plants, production can significantly exceed Swiss consumption.
If the energy surpluses of the Swiss energy supply system have to be absorbed by storage systems without international exchange, an appropriate storage design is necessary. The theoretical storage power and capacity (energy) required in addition to storage at distribution grid level is shown in Table 5‑9. In this context, a purely national approach would result in a massive storage requirement at system level in 2050 for the C&E and E supply variants under consideration. Storage facilities with a storage capacity of up to 680 GWh and a storage power of about 8000 MW would be required to cover all surpluses in Switzerland. The very high values of the isolated consideration of Switzerland are particularly due to the above-mentioned energy surpluses in the low load periods of summer.
An extension of the analysis to include transmission capacities to adjacent electricity supply systems offers potential for reducing the storage requirements of the Swiss system. For this purpose, Table 5‑9 lists the storage parameters of the storage systems at system level, in order to be able to store surpluses that would have to be curtailed due to a lack of national and international demand. A considerable reduction in storage requirements at system level can be observed by including exchange capacities with neighboring countries. Simultaneously high production from wind and PV and the minimum production volumes of, for example, geothermal energy and run-of-the-river hydroelectric power plants can cause long periods of surplus within Switzerland. The duration and quantity of surplus energy can be reduced by using export capacities, since neighboring electricity supply systems are available for the exchange of supply-dependent production quantities due to different production structures and weather conditions. This results in a required storage power in the range of almost 7000 MW for the E supply variants, while the storage capacity would have to be 62 GWh to absorb all Swiss surpluses.
Table 5‑9: Theoretical storage requirements at system level without and with cross-border exchange (Energiespeicher in der Schweiz, BFE 2013)
national only | with exchange | reduction by exchange | ||||
Power [MW] | Capacity [GWh] | Power [MW] | Capacity [GWh] | Power [%] | Capacity [%] | |
Scenario NEP-E | 8025 | 680 | 6850 | 62 | 15 | 90 |
However, the question of the dimensioning of the storage parameters arises, if not the entire surplus has to be stored. Due to the diversity of surplus events at system level, the premises used for the distribution grid level are no longer valid here. Storage of all surpluses still does not seem reasonable. An analysis of the storage capacity that makes sense from an economic point of view cannot be made plausible without model-based optimising at system level. Therefore, we refer at this point to the results on the profitability of the storage power plants, which are further examined in Module C of the presented study (Hewicker et al. 2013).
Large-scale storage systems are suitable for storing energy surpluses of the overall system. However, to store the system surpluses in the NEP/C&E and POM/C&E scenarios, additional storage capacities are required that exceed the sum of the currently installed and planned pumped storage capacities. The obvious approach here is to include the storage capacities of the large storage reservoirs of seasonal storage. The currently installed and planned Swiss pumped storage plants have a typical storage capacity that allows a discharge duration in the range of 12 hours. Storage of longer-term surpluses requires a much higher storage capacity. In principle, the storage reservoirs could meet this requirement. However, in order for surpluses to be stored, firstly, the usability of the storage reservoirs would have to be verified on the basis of an analysis of the annual development of the filling levels and the surpluses that occur, and secondly, the power plants of the reservoirs would have to be equipped with a pumping system and existing reservoirs would have to be extended or new reservoirs built at great expense.
Power-to-gas is the only technology presented here that offers the prospect of a seasonal large-scale storage facility. However, short-term storage is also possible (e.g. in the weekly range). In terms of storage capacity, significantly larger classes are feasible than for pumped storage or compressed air storage, as gas storage facilities already in use today can be used.